Renewable and Sustainable Energy Thermodynamics
In the face of escalating environmental challenges and a growing global demand for clean energy, the study of thermodynamics takes on renewed significance. Within this field, renewable and sustainable energy thermodynamics examines how energy from natural sources can be harnessed and transformed efficiently, with minimal ecological impact. Grounded in fundamental principles from science and physics, this area connects theoretical insight with pressing real-world applications.
The shift toward sustainability requires a deep understanding of how systems evolve under thermal gradients, governed by the laws of thermodynamics. Students are encouraged to revisit foundational concepts from classical thermodynamics and apply them to the operation of thermodynamic cycles commonly used in power generation. From Carnot engines to Rankine and Brayton cycles, thermodynamic models help assess efficiency limits and point the way toward sustainable optimization.
Incorporating principles from engineering thermodynamics, this domain evaluates the performance of solar collectors, geothermal plants, biomass combustion systems, and wind-driven turbines. With the support of heat transfer analysis, students explore how to reduce thermal losses and enhance energy capture. Meanwhile, tools from computational thermodynamics enable advanced modeling of renewable systems under real-world constraints.
The topic also overlaps with statistical thermodynamics, which provides a molecular perspective on entropy, temperature, and energy distributions—key to analyzing efficiency and disorder in complex systems. For dynamic systems far from equilibrium, insights from non-equilibrium thermodynamics are vital, especially in managing intermittent sources like solar and wind energy.
In addition to thermodynamic expertise, the development of sustainable energy technologies benefits from interdisciplinary knowledge. Concepts in physical chemistry play a role in battery storage and fuel cell design. Likewise, advances in thermodynamics of materials help optimize phase-change media and heat-resistant coatings. Related ideas from phase equilibria and transitions support the analysis of energy storage mechanisms, such as latent heat systems.
At the cutting edge, research intersects with plasma physics and nuclear fusion, where breakthroughs could redefine long-term energy sustainability. Understanding fusion systems also requires familiarity with nuclear reactions and the nature of radioactive isotopes. Even concepts such as atomic structure, wave-particle duality, and wave functions provide the theoretical underpinnings for harnessing energy at microscopic scales.
Finally, the thermodynamics of sustainability cannot be divorced from broader physical frameworks like relativity and electrodynamics, which are essential in modeling large-scale systems and high-energy interactions. Taken together, these interconnected disciplines help students envision not only how energy works, but how it can work for the future.
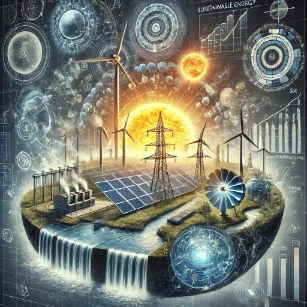
Table of Contents
Core Concepts in Renewable and Sustainable Energy Thermodynamics
The First and Second Laws of Thermodynamics in Renewable Energy
The First Law of Thermodynamics states that energy cannot be created or destroyed, only transformed from one form to another. This principle is fundamental in renewable energy systems, where solar panels convert radiant energy into electrical power, and wind turbines transform kinetic energy into mechanical work. The Second Law of Thermodynamics, which deals with entropy, highlights the inefficiencies in energy conversion, dictating the maximum possible efficiency of these systems. For example, heat engines used in geothermal and biomass energy production must adhere to thermodynamic limitations, making efficiency improvements critical in sustainable energy design.
Energy Conversion and Storage in Renewable Systems
Renewable energy systems depend on efficient energy conversion and storage to balance supply and demand. Technologies such as batteries, pumped hydro storage, hydrogen fuel cells, and thermal energy storage are designed to manage intermittent energy availability. Thermodynamics governs the performance of these storage systems by analyzing energy losses, heat dissipation, and conversion efficiency. For instance, in lithium-ion batteries, thermodynamic modeling helps optimize chemical reactions to improve longevity and performance.
Exergy Analysis and Sustainability Metrics
Exergy analysis is a key thermodynamic tool used to assess the quality and usability of energy in renewable systems. Unlike energy, which is conserved, exergy measures the work potential of a given energy source. In renewable energy systems, exergy analysis helps identify inefficiencies and potential improvements. Sustainability metrics, such as carbon footprint, energy return on investment (EROI), and life cycle assessment (LCA), further evaluate the long-term feasibility of energy technologies.
Thermodynamics of Heat Transfer in Renewable Energy
Heat transfer plays a crucial role in many renewable energy applications, particularly in solar thermal, geothermal, and biomass systems. Understanding conduction, convection, and radiation enables engineers to design efficient solar collectors, heat exchangers, and thermal storage systems. For example, concentrating solar power (CSP) plants rely on thermodynamic principles to optimize heat capture and conversion into electricity. Similarly, geothermal power plants harness Earth’s heat by optimizing fluid flow and heat extraction processes.
Focus in Renewable and Sustainable Energy Thermodynamics
One of the primary research focuses in renewable energy thermodynamics is improving energy efficiency and reducing waste. Scientists work on increasing the conversion efficiency of solar panels, wind turbines, and thermal energy systems by minimizing losses due to heat dissipation, aerodynamic drag, and electrical resistance. Innovations in nanomaterials, advanced coatings, and optimized system designs contribute to more sustainable energy solutions.
Another significant focus is integrating renewable energy with smart grids and hybrid systems. By combining multiple energy sources, such as solar and wind power with battery storage and hydrogen fuel cells, researchers develop systems that balance energy supply and demand. Thermodynamics plays a vital role in analyzing how these hybrid systems operate efficiently, ensuring a stable, scalable, and resilient power infrastructure.
How the Study of Renewable and Sustainable Energy Thermodynamics Helps Prepare Students for University Studies
Studying Renewable and Sustainable Energy Thermodynamics provides students with a strong foundation in engineering thermodynamics, energy systems, and sustainability science. At the university level, these principles are crucial for coursework in mechanical engineering, environmental engineering, power generation technology, and physics. Understanding thermodynamic concepts enables students to analyze and design efficient energy conversion processes, preparing them for research and careers in sustainable energy development.
Core Topics Covered to Help Prepare for University Studies in Renewable and Sustainable Energy Thermodynamics
Students studying this field typically cover essential topics such as:
- Thermodynamic laws and their application in energy systems
- Energy conversion and storage technologies
- Exergy analysis and system efficiency optimization
- Heat transfer in renewable energy applications
- Sustainability assessment and environmental impact analysis
Mastering these areas equips students with problem-solving skills and analytical tools to tackle real-world challenges in renewable energy engineering and sustainable development.
Common Challenges Faced in the Study of Renewable and Sustainable Energy Thermodynamics
One major challenge in studying renewable energy thermodynamics is understanding the limitations imposed by thermodynamic laws on energy conversion. Students often struggle with concepts like entropy, exergy loss, and the Carnot efficiency limit, which determine the theoretical performance of energy systems.
Another challenge is modeling and simulation. Renewable energy systems are highly variable due to weather patterns, geographic constraints, and seasonal fluctuations, making thermodynamic analysis more complex. Students must learn to work with computational tools to model and optimize real-world energy applications.
Examples of Current Applications of Renewable and Sustainable Energy Thermodynamics in Society
- Solar Power: Thermodynamics governs the efficiency of solar panels and concentrated solar power (CSP) plants, which generate electricity from sunlight.
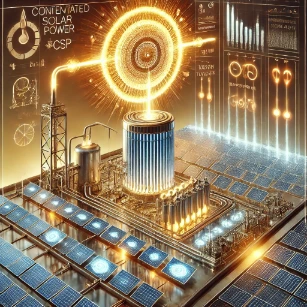
- Wind Energy: Aerodynamic and thermodynamic principles optimize wind turbine blade design for maximum energy extraction.
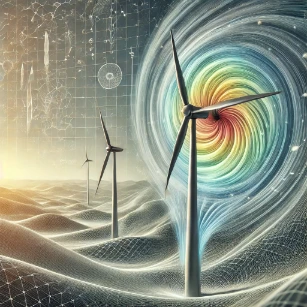
- Geothermal Energy: Heat transfer and fluid thermodynamics help in designing geothermal power plants that extract and utilize underground heat.
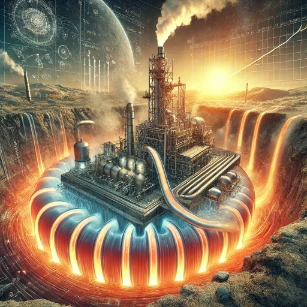
- Hydropower: Hydroelectric plants use fluid dynamics and energy conversion principles to generate electricity efficiently.
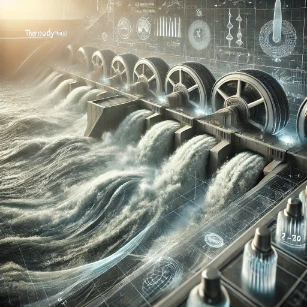
Examples of Emerging Applications of Renewable and Sustainable Energy Thermodynamics in Society
- Hydrogen Economy: Thermodynamics plays a key role in optimizing hydrogen production, storage, and fuel cell technologies for zero-emission energy solutions.
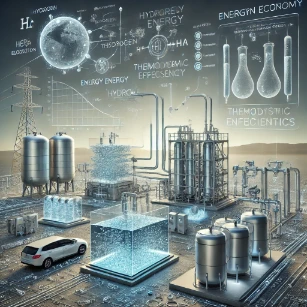
- Artificial Photosynthesis: Inspired by natural processes, researchers are developing systems that use solar energy to convert water and carbon dioxide into synthetic fuels.
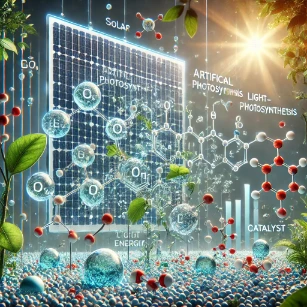
- Phase-Change Thermal Storage: New materials that store and release heat efficiently are being integrated into renewable energy systems to provide continuous power supply.
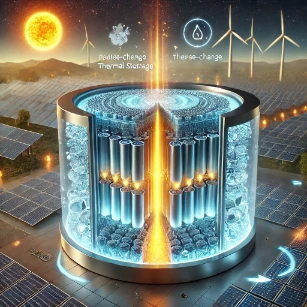
- Waste Heat Recovery: Advances in thermoelectric generators and organic Rankine cycles allow industries to capture and reuse waste heat, improving sustainability.
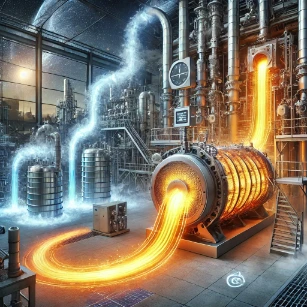
Why Study Renewable and Sustainable Energy Thermodynamics
Supporting a Sustainable Energy Future
Renewable energy thermodynamics applies classical and modern principles to clean energy systems. Students learn how solar, wind, geothermal, and bioenergy processes are governed by thermodynamic laws. Understanding these systems supports innovation in sustainable technology. It prepares students to address global environmental challenges.
Efficiency and Resource Management
Students analyze the thermodynamic limits of renewable energy devices such as solar panels and fuel cells. They evaluate the efficiency of energy conversion, storage, and transmission. This fosters critical thinking about resource optimization and life-cycle analysis. It builds awareness of the trade-offs in sustainable design.
Thermodynamics of Energy Storage
Energy storage is key to balancing supply and demand in renewable systems. Students study thermodynamic principles behind batteries, hydrogen storage, and thermal energy reservoirs. These insights support design of resilient and efficient storage solutions. They ensure reliability and performance in energy networks.
Modeling and System Integration
Students learn to model renewable systems using first and second law analysis. They integrate thermodynamics with fluid flow, mass transfer, and environmental data. This prepares them to design hybrid and distributed energy systems. It supports holistic and systems-level thinking.
Ethical and Global Context
Studying renewable thermodynamics cultivates an understanding of equity, environmental justice, and climate responsibility. Students explore how energy access and sustainability intersect. This encourages them to design inclusive and globally mindful solutions. It empowers them to lead in a transitioning energy landscape.
Renewable and Sustainable Energy Thermodynamics Conclusion
The study of Renewable and Sustainable Energy Thermodynamics is essential for advancing clean energy technologies and addressing the global energy crisis. By understanding thermodynamic principles, scientists and engineers develop efficient, scalable, and environmentally friendly energy systems. As research in this field progresses, new breakthroughs in hydrogen energy, energy storage, and waste heat recovery will continue to shape the future of sustainable energy. Students and professionals entering this discipline will play a critical role in reducing carbon emissions, improving energy efficiency, and building a sustainable energy future.
Renewable and Sustainable Energy Thermodynamics: Review Questions and Answers:
1. What is renewable and sustainable energy thermodynamics?
Answer: It is the study of energy transformations and efficiencies in systems that utilize renewable resources, applying thermodynamic principles to optimize energy conversion and minimize environmental impact.
2. How does the First Law of Thermodynamics apply to renewable energy systems?
Answer: The First Law, which states energy is conserved, ensures that the energy input from renewable sources is accounted for in the output work and internal energy changes, guiding system efficiency and design.
3. What role does entropy play in sustainable energy processes?
Answer: Entropy measures disorder in a system and dictates the irreversibility of energy conversion processes. In sustainable energy, minimizing entropy production is key to improving system efficiency and reducing waste heat.
4. How can thermodynamic analysis improve the design of renewable energy devices?
Answer: Thermodynamic analysis helps predict performance, optimize operating conditions, and identify losses in devices like solar panels, wind turbines, and geothermal systems, leading to enhanced efficiency and sustainability.
5. What is the significance of the Carnot cycle in evaluating renewable energy systems?
Answer: The Carnot cycle provides the theoretical maximum efficiency for a heat engine, serving as a benchmark to assess and improve the performance of practical renewable energy conversion systems.
6. How do phase change materials (PCMs) relate to sustainable energy thermodynamics?
Answer: PCMs absorb and release latent heat during phase transitions, making them effective for thermal energy storage and regulation in renewable energy systems, thereby improving efficiency and stability.
7. What is exergy analysis and why is it important in renewable energy applications?
Answer: Exergy analysis quantifies the usable work potential of energy within a system. It identifies inefficiencies and areas for improvement, which is crucial for optimizing renewable energy systems and reducing environmental impact.
8. How does the Second Law of Thermodynamics influence the efficiency of renewable energy systems?
Answer: The Second Law limits the maximum efficiency achievable by any energy conversion process due to inevitable entropy generation, guiding engineers to design systems that approach these theoretical limits.
9. In what ways do renewable energy systems benefit from computational thermodynamics?
Answer: Computational thermodynamics uses simulations to model complex energy conversion processes, enabling the design, optimization, and scaling of renewable energy systems with improved performance and reduced costs.
10. How do sustainable energy strategies incorporate thermodynamic principles to reduce environmental impact?
Answer: They apply thermodynamic concepts to enhance energy conversion efficiency, recover waste heat, and design processes that minimize energy losses, leading to cleaner, more sustainable operations in power generation and industrial applications.
Renewable and Sustainable Energy Thermodynamics: Thought-Provoking Questions and Answers
1. How could breakthroughs in renewable energy thermodynamics revolutionize global energy markets?
Answer: Advances could lead to highly efficient, low-emission energy systems that reduce reliance on fossil fuels. Improved thermodynamic cycles and energy recovery methods may lower costs, drive widespread adoption of renewables, and reshape global energy policies toward sustainability.
2. In what ways can exergy analysis transform the design of next-generation renewable energy systems?
Answer: Exergy analysis pinpoints where energy degradation occurs. By minimizing these losses, designers can develop systems that maximize usable energy output, resulting in more efficient solar, wind, or geothermal installations with lower environmental footprints.
3. How might integrating phase change materials with renewable technologies impact energy storage solutions?
Answer: Integrating PCMs can enhance energy storage by maintaining stable temperatures and releasing or absorbing heat during phase transitions. This can improve the performance and reliability of renewable systems, particularly in solar thermal and building applications.
4. What are the challenges in applying the Carnot efficiency limit to real-world renewable energy systems?
Answer: Real systems face irreversibilities such as friction, heat loss, and material imperfections that prevent them from reaching the ideal Carnot limit. Overcoming these challenges requires innovative materials, advanced designs, and effective waste heat recovery strategies.
5. How can computational modeling be used to optimize energy conversion in renewable systems?
Answer: Computational modeling simulates the intricate processes of heat transfer, fluid dynamics, and chemical reactions in energy systems. This allows engineers to test various configurations, predict system performance, and optimize designs before physical implementation, saving time and resources.
6. What implications does the Second Law of Thermodynamics have for the future of sustainable energy production?
Answer: The Second Law sets a fundamental limit on energy conversion efficiency. Understanding these limits helps in designing systems that minimize energy loss, promoting the development of technologies that approach ideal efficiency and reduce environmental impact.
7. How might advances in nanotechnology and thermodynamics lead to breakthroughs in renewable energy devices?
Answer: Nanotechnology can improve material properties such as thermal conductivity and catalytic activity. When combined with thermodynamic principles, these advances may lead to more efficient solar cells, batteries, and fuel cells, significantly enhancing renewable energy performance.
8. How do temperature fluctuations affect the performance and reliability of renewable energy systems?
Answer: Temperature fluctuations can cause thermal expansion, stress, and inefficiencies in energy systems. Understanding these effects through thermodynamic analysis can lead to better insulation, adaptive control systems, and materials that maintain performance under varying conditions.
9. What role does sustainability play in the thermodynamic analysis of energy systems?
Answer: Sustainability in thermodynamic analysis focuses on maximizing energy efficiency, minimizing waste, and reducing environmental impact. It involves designing systems that not only conserve energy but also utilize renewable resources and reduce greenhouse gas emissions.
10. How could improved heat transfer techniques enhance the efficiency of renewable energy systems?
Answer: Enhanced heat transfer techniques can reduce thermal resistance and improve energy exchange rates. This leads to more effective cooling and heating in devices, better energy conversion in power cycles, and overall improved efficiency of renewable energy installations.
11. In what ways can interdisciplinary research between thermodynamics and environmental science contribute to climate change mitigation?
Answer: Interdisciplinary research can optimize energy systems, improve waste heat management, and design low-emission technologies. By integrating thermodynamic principles with environmental impact studies, researchers can develop sustainable solutions that address both energy needs and climate change challenges.
12. What future innovations in computational thermodynamics might lead to breakthroughs in renewable energy system design?
Answer: Future innovations could include advanced simulation algorithms, machine learning-enhanced models, and real-time monitoring systems. These tools will allow for precise optimization of energy flows, phase transitions, and system efficiency, ultimately leading to more robust and cost-effective renewable energy technologies.
Renewable and Sustainable Energy Thermodynamics: Numerical Problems and Solutions
1. Calculate the energy of a photon with a wavelength of 600 nm using E = hc/λ. (h = 6.626×10⁻³⁴ J·s, c = 3.0×10⁸ m/s)
Solution:
λ = 600 nm = 600×10⁻⁹ m
E = (6.626×10⁻³⁴ × 3.0×10⁸) / (600×10⁻⁹)
≈ 1.9878×10⁻²⁵ J·m / 600×10⁻⁹ m
≈ 3.313×10⁻¹⁹ J.
2. For a reversible process, if 500 J of heat is absorbed at 350 K, calculate the change in entropy ΔS.
Solution:
ΔS = Q/T = 500 J / 350 K ≈ 1.429 J/K.
3. Calculate the Carnot efficiency for a heat engine operating between 900 K and 300 K.
Solution:
η = 1 – T_c/T_h = 1 – 300/900
= 1 – 0.3333 = 0.6667 or 66.67%.
4. Determine the work done during an isothermal expansion of 1 mole of an ideal gas at 400 K from 1.0 L to 3.0 L. (R = 8.314 J/(mol·K), 1 L = 0.001 m³)
Solution:
W = nRT ln(V_f/V_i)
= 1×8.314×400×ln(3.0/1.0)
= 3325.6×ln(3)
≈ 3325.6×1.0986
≈ 3653 J.
5. If a system undergoes a process where it absorbs 1200 J of heat and performs 800 J of work, calculate the change in internal energy ΔU.
Solution:
ΔU = Q – W = 1200 J – 800 J = 400 J.
6. A substance requires 100 kJ of energy to melt 2.5 kg. Calculate the latent heat of fusion in kJ/kg.
Solution:
Latent heat = Total energy / Mass = 100 kJ / 2.5 kg = 40 kJ/kg.
7. For a reaction with ΔH = -60 kJ/mol and ΔS = -150 J/(mol·K) at 350 K, calculate ΔG.
Solution:
ΔG = ΔH – TΔS
= (-60,000 J/mol) – 350×(-150 J/(mol·K))
= -60,000 + 52,500
= -7,500 J/mol.
8. Calculate the equilibrium constant K at 350 K for a reaction with ΔG = -8 kJ/mol. (R = 8.314 J/(mol·K))
Solution:
ΔG = -RT ln K
-8000 J/mol = -8.314×350 ln K
ln K = 8000 / (8.314×350)
≈ 8000 / 2909.9 ≈ 2.747
K = e^(2.747) ≈ 15.6.
9. For a system with a partition function Z = 150 at T = 300 K, calculate the Helmholtz free energy F using F = -k_BT ln Z. (k_B = 1.38×10⁻²³ J/K)
Solution:
F = -1.38×10⁻²³×300×ln(150)
ln(150) ≈ 5.0106
F ≈ -1.38×10⁻²³×300×5.0106
≈ -2.077×10⁻²⁰ J.
10. In a simulation with 4×10⁶ iterations, each taking 1.5×10⁻⁵ s, calculate the total simulation time.
Solution:
Total time = 4×10⁶ × 1.5×10⁻⁵ s = 60 s.
11. If a phase change requires 95 kJ of heat to melt 2 kg of a substance, calculate the latent heat of fusion in kJ/kg.
Solution:
Latent heat = 95 kJ / 2 kg = 47.5 kJ/kg.
12. A heat exchanger transfers 3000 J of energy from a hot fluid at 550 K to a cold fluid at 350 K. Calculate the total entropy change for the process (assuming reversible transfer).
Solution:
Entropy change for hot fluid: ΔS_hot = -3000 J / 550 K ≈ -5.455 J/K
Entropy change for cold fluid: ΔS_cold = 3000 J / 350 K ≈ 8.571 J/K
Total ΔS = -5.455 + 8.571 ≈ 3.116 J/K.