Science
Science is a dynamic and continually evolving field that seeks to explain the natural world through observation, experimentation, and critical reasoning. At the heart of this exploration lies Biology, a discipline that investigates living organisms and their interactions with the environment. The microscopic world of life is unraveled through Cell Biology, which introduces students to the fundamentals of cell structure and the processes of cell cycle and cell development. Understanding cell communication and cell physiology forms a foundation for grasping more complex biological systems.
Explorations into how organisms interact with one another and their environments are found in Ecology, while the origins and adaptations of species over time are addressed in Evolutionary Biology. A deeper understanding of heredity and traits is achieved through Genetics, spanning the classical principles of Mendelian Genetics to cutting-edge applications in Molecular Genetics.
The structure and function of genetic material are explored in topics such as DNA and RNA, as well as the intricacies of gene expression and genetic mutation. Learners gain insights into the molecular basis of inheritance and examine how these processes influence health through applications of genetics in medicine.
Technological advancements are reflected in the study of DNA technology and the role of protein synthesis in cellular functions. Additionally, students are introduced to molecular techniques in research, which serve as tools for advancing biomedical innovation. These scientific approaches are essential for understanding the evolutionary processes covered in molecular evolution.
The scope of science broadens further through the study of variation in populations, as seen in population genetics and quantitative genetics. To understand organisms at the genome level, learners delve into genomics, which integrates bioinformatics and large-scale data analysis to map genetic landscapes. Together, these domains demonstrate the richness and interconnectivity of scientific inquiry, fostering a mindset of curiosity and evidence-based reasoning essential for students preparing for university-level science and beyond.
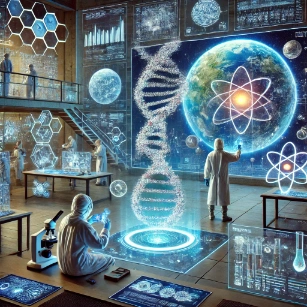
Table of Contents
Physics
Focus: The study of matter, energy, and the fundamental laws of the universe.
Physics is the foundational science that seeks to understand the behavior of the universe at every scale—from the subatomic world to the vastness of galaxies. It explores how matter and energy interact, how forces govern motion, and how the fabric of space and time shapes all physical phenomena. Whether applied in technological innovation, theoretical research, or experimental science, physics enables deeper insight into the workings of nature and supports engineering, medicine, computing, environmental science, and many other domains.
- Subfields:
- Classical Mechanics: Examines the motion of bodies under the influence of forces and torques. This includes Newton’s laws of motion, energy conservation, linear and angular momentum, and the dynamics of rigid bodies and fluids. Classical mechanics is crucial in engineering disciplines and forms the basis for designing machines, vehicles, and structures.
- Thermodynamics: Explores the relationships between heat, work, energy, and entropy. Thermodynamics governs everything from heat engines and refrigeration cycles to the behavior of gases and phase transitions. Its principles are foundational in chemistry, biology, and environmental science as well.
- Electricity and Magnetism: Investigates electric fields, magnetic fields, circuits, and electromagnetic waves. Applications include motors, generators, wireless communication, and power distribution systems. This field also laid the groundwork for the unification of light and electromagnetism through Maxwell’s equations.
- Lights and Optics: Focuses on the behavior of light, its interaction with matter, and the wave-particle duality of photons. Optics is crucial in technologies such as cameras, microscopes, fiber optics, and laser systems, and underpins the development of modern imaging and communication tools.
- Modern Physics: Studies the non-classical phenomena that emerge at very small or very fast scales. Topics include atomic physics, nuclear physics, and particle physics, which delve into the structure of atoms and the fundamental particles of nature. This subfield has revolutionized understanding through quantum mechanics and relativity.
- Astrophysics: Investigates celestial phenomena and the structure of the cosmos, including stars, black holes, galaxies, and cosmological events. Through astrophysics, physicists study the origins of the universe, the nature of dark matter and dark energy, and the fate of cosmic structures. See related focus on black holes.
- Applications:
- Development of transformative technologies such as lasers, semiconductors, and nuclear reactors. Physics has enabled the digital revolution, from transistors to quantum computing, influencing the design of everything from smartphones to particle accelerators.
- Driving advancements in space exploration, including the design of propulsion systems, satellite trajectories, and life-support systems. Applications span from GPS and weather forecasting to interplanetary missions.
- Enhancing our understanding of climate systems, through radiative transfer models, fluid dynamics, and thermodynamics, which are key to environmental monitoring and sustainability initiatives.
- Providing diagnostic and therapeutic tools in medicine—such as MRI, PET scans, radiation therapy, and ultrasound—based on principles of nuclear physics and wave propagation.
Ongoing research in theoretical and experimental physics not only deepens our understanding of the universe but also leads to practical innovations that benefit society. Institutions like CERN serve as global hubs for such exploration, uniting physicists from around the world to investigate fundamental particles, forces, and the early moments of the universe.
Chemistry
Focus: The study of substances, their properties, reactions, and how they interact.
Chemistry is the central science that bridges physics, biology, and environmental studies by examining the composition, structure, behavior, and transformation of matter. From atoms and molecules to complex materials and biochemical pathways, chemistry provides the theoretical and experimental tools necessary to explore the building blocks of the universe. It informs the development of new materials, fuels innovation in pharmaceuticals, and plays a pivotal role in sustainability and global health.
- Subfields:
- Organic Chemistry: Focuses on the study of carbon-containing compounds and their reactions. Organic chemistry underpins the synthesis of drugs, polymers, dyes, agrochemicals, and biomolecules. From aspirin and plastics to DNA and natural products, organic reactions form the basis for many technologies in medicine, materials science, and biotechnology.
- Inorganic Chemistry: Studies a broad range of substances that do not rely on carbon-hydrogen frameworks, including metals, salts, minerals, and coordination complexes. It plays a crucial role in catalysis, nanomaterials, pigment production, and electronic components such as semiconductors and superconductors.
- Physical Chemistry: Applies principles of physics to explain chemical systems. It focuses on reaction kinetics, thermodynamics, quantum chemistry, and molecular dynamics. This subfield supports research in electrochemistry, spectroscopy, and statistical mechanics and contributes significantly to understanding chemical equilibria and energy transformations.
- Analytical Chemistry: Involves identifying the composition of substances through qualitative and quantitative techniques. Methods include chromatography, spectroscopy, and electroanalytical techniques, which are vital in quality control, forensics, clinical diagnostics, and environmental testing.
- Biochemistry: Explores the chemical processes and substances that occur within living organisms. It provides insight into enzymes, metabolism, genetic expression, and cellular communication. Biochemistry forms the basis of molecular biology, biotechnology, and modern medicine.
- Environmental Chemistry: Examines the chemical composition of air, water, and soil, as well as the transformation and fate of pollutants in the environment. It addresses topics such as acid rain, smog formation, heavy metal contamination, and the role of climate change in chemical cycles like carbon and nitrogen.
- Industrial Chemistry: Focuses on scaling up chemical reactions and designing large-scale production methods for materials such as polymers, fuels, fertilizers, and specialty chemicals. It ensures efficient and safe chemical processing, often with emphasis on green chemistry and waste minimization.
- Applications:
- Drug development in the pharmaceutical industry: Chemistry guides every stage from molecular design and synthesis to clinical trials and production. Advances in medicinal chemistry have produced antiviral drugs, antibiotics, anticancer agents, and vaccines that save millions of lives.
- Creation of sustainable materials and chemical processes: Chemists are at the forefront of developing biodegradable plastics, recyclable materials, and efficient catalytic processes that reduce industrial emissions and waste.
- Environmental monitoring and pollution control: Analytical techniques are used to detect trace contaminants in water, soil, and air, enabling regulatory enforcement and the design of remediation strategies.
- Enhancing agricultural productivity through fertilizers, pesticides, and soil conditioning agents while minimizing ecological harm and supporting food security.
- Advancing nanotechnology, where chemistry contributes to the design of nanomaterials with tailored properties for use in medicine, energy, and computing.
- Supporting clean energy transitions by developing materials for fuel cells, solar panels, hydrogen storage, and efficient batteries. Organizations such as RSC’s Chemistry World highlight breakthroughs in hydrogen chemistry and green alternatives.
Overall, chemistry equips students and researchers with a versatile framework for innovation, problem-solving, and critical thinking. It is central to tackling challenges in climate change, healthcare, energy, and sustainable development.
Biology
Focus: The study of living organisms, their structures, functions, and interactions.
Biology is a foundational life science that explores the complexities of life at multiple levels—from molecular processes within cells to the interdependence of organisms in ecosystems. It integrates knowledge from chemistry, physics, and environmental science to address key questions about growth, development, reproduction, evolution, and the sustainability of life. By understanding how organisms function and adapt, biology contributes to medical advancements, agricultural resilience, environmental protection, and the development of biotechnology. It is essential for addressing global challenges such as disease outbreaks, food security, and climate change impacts on biodiversity.
- Subfields:
- Cell Biology: Examines the structure, function, and processes within cells—the basic units of life. Topics include cellular respiration, mitosis and meiosis, organelle dynamics, membrane transport, and intracellular signaling mechanisms. Cell biology underpins biotechnology, cancer research, regenerative medicine, and immunology.
- Physiology: Focuses on the physical and biochemical processes that sustain living organisms. It investigates how systems such as the nervous, respiratory, circulatory, digestive, and endocrine systems maintain homeostasis and adapt to environmental challenges in plants, animals, and humans.
- Ecology: Studies the relationships between organisms and their environments. It explores population dynamics, community interactions, ecosystem services, and biodiversity conservation. Topics include food webs, nutrient cycles, ecological succession, and the effects of climate change on ecosystems.
- Genetics: Investigates how traits are inherited through genes, exploring DNA structure, gene expression, mutation, and modern tools like CRISPR. Genetics is crucial in fields such as genomics, personalized medicine, and crop improvement.
- Evolutionary Biology: Explores how species adapt and diversify over time through mechanisms like natural selection, genetic drift, and speciation. Evolutionary theory forms the basis for understanding phylogenetic relationships, antibiotic resistance, and the origins of life.
- Applications:
- Innovations in healthcare: Biology has enabled breakthroughs in gene therapy, vaccine development, monoclonal antibodies, organ transplantation, and regenerative medicine. Understanding viral biology and immune responses was key to the rapid development of mRNA vaccines.
- Conservation and biodiversity protection: Ecologists and biologists develop strategies for preserving endangered species, restoring habitats, and maintaining ecological balance. Conservation genetics helps track population health and mitigate the effects of habitat fragmentation.
- Agricultural advancements: Biotechnology enables the creation of genetically modified organisms (GMOs) with traits such as pest resistance, drought tolerance, and enhanced nutritional value. Synthetic biology offers new solutions in sustainable farming and food production.
- Climate change mitigation: Understanding plant biology and carbon cycles allows scientists to explore carbon sequestration, develop resilient crops, and design climate-smart agricultural practices.
- Bioinformatics and computational biology: Leveraging big data and artificial intelligence, researchers map genomes, model protein structures, and study complex networks in systems biology. Tools like NCBI’s GenBank provide access to extensive biological databases for global collaboration.
As a discipline, biology not only helps us understand life’s inner workings but also empowers innovation in medicine, sustainability, and technology. With increasing integration across disciplines, biology will continue to drive transformative solutions in the 21st century.
Earth Sciences
Focus: The study of Earth’s physical characteristics, processes, and history.
Earth Sciences encompass the comprehensive study of our planet’s structure, natural systems, and evolutionary timeline. It is an interdisciplinary field that combines geology, meteorology, oceanography, and paleontology to understand dynamic processes such as plate tectonics, erosion, climate change, and the hydrological cycle. By examining both current phenomena and the Earth’s geological past, scientists in this domain provide critical insights into natural hazards, resource management, and environmental sustainability. The discipline plays an essential role in informing policy decisions related to disaster risk reduction, climate action, and sustainable development.
- Subfields:
- Geology: Geology is the foundation of Earth Sciences, investigating the Earth’s internal and surface structures, mineral composition, and geodynamic activities. It covers tectonic plate movements, volcanic eruptions, earthquake mechanisms, rock formation, sedimentation, and stratigraphy. Applied geology supports earthquake engineering, petroleum exploration, groundwater mapping, and construction site assessments.
- Meteorology: This subfield focuses on the study of the atmosphere, atmospheric pressure systems, wind patterns, humidity, and precipitation. Meteorologists use data from satellites, radar, and weather balloons to predict short-term and long-term weather phenomena, helping in early warning systems for storms, floods, and extreme weather events. Meteorology is also central to understanding climate variability and atmospheric pollution.
- Oceanography: Oceanography examines ocean currents, salinity, tides, and their effects on marine biodiversity, coastal systems, and global climate. Physical oceanographers model currents and sea-level rise; biological oceanographers study food webs and ecosystems; geological oceanographers analyze seabed structures; and chemical oceanographers investigate salinity, oxygenation, and pollution. The field is vital for managing fisheries, tracking oil spills, and studying the role of oceans in carbon storage.
- Paleontology: Paleontology reconstructs Earth’s biological history through fossil evidence. This field sheds light on extinction events, evolutionary transitions, and the age of geological formations. It intersects with evolutionary biology, anthropology, and geology, and is crucial in dating rock strata, locating oil reserves, and understanding long-term ecological changes.
- Applications:
- Natural disaster prediction and mitigation: Earth scientists use seismology, remote sensing, and geological hazard mapping to assess earthquake risk zones, predict volcanic eruptions, and develop early warning systems for landslides and tsunamis. Technologies like LIDAR and InSAR allow precise monitoring of land surface deformations to inform infrastructure resilience.
- Exploration of natural resources: Earth Science supports the sustainable extraction of minerals, metals, oil, and gas through geophysical surveys, stratigraphic analysis, and modeling subsurface geology. Hydrogeology is employed to locate and manage groundwater, which is critical in arid regions. Mining geologists assess ore body structures and evaluate environmental impact.
- Climate modeling and environmental monitoring: Climate scientists create sophisticated models using paleoclimate data, greenhouse gas measurements, and feedback loop simulations to project future climate scenarios. These models guide global policies on emissions, adaptation, and sustainability. Tools like Earth observation satellites and GIS platforms enable global-scale environmental analysis.
- Urban and regional planning: Geologists and geographers contribute to land-use planning by identifying fault lines, floodplains, and erosion zones. Their work helps avoid hazardous areas during urban development and supports the design of more resilient cities.
- Education and outreach: Earth Science plays a pivotal role in public education on sustainability, natural hazards, and planetary stewardship. It is also integral to promoting scientific literacy and inspiring careers in geoscience and environmental management.
- Global collaboration: Institutions such as the U.S. Geological Survey (USGS) contribute open-access datasets, hazard maps, and scientific research, enabling countries worldwide to benefit from shared knowledge and best practices in Earth system science.
As humanity grapples with environmental degradation, resource scarcity, and climate volatility, Earth Sciences provide the tools to understand, adapt to, and mitigate these challenges. The field’s integration with data science, AI, and remote sensing will further enhance its capacity to drive sustainable development and protect life on Earth.
Environmental Science
Focus: Addressing ecological and sustainability challenges through interdisciplinary approaches.
Environmental Science is an integrative discipline that combines knowledge from biology, chemistry, physics, geology, policy studies, and social sciences to understand the complex interactions between human activity and the natural world. This field equips students and researchers with the tools to diagnose environmental problems, develop mitigation strategies, and promote sustainable solutions for global and local ecosystems. Environmental scientists analyze data from air, water, and soil; model climate and ecological systems; and advocate for policies that promote renewable energy, conservation, pollution control, and sustainable development. As humanity faces increasing environmental crises, this discipline is essential for building a sustainable future and fostering environmental stewardship.
- Subfields:
- Conservation Science: Focuses on preserving biodiversity and protecting ecosystems from degradation. Conservation scientists work to maintain genetic diversity, restore habitats, and implement protected area management. Techniques include habitat corridors, wildlife tracking, ecosystem service valuation, and invasive species control. Their work supports both ecological integrity and indigenous community livelihoods.
- Renewable Energy Studies: Explores the science and engineering behind sustainable energy sources such as solar photovoltaics, wind turbines, geothermal systems, and biofuels. This subfield assesses environmental impact, life-cycle efficiency, and energy policy. Researchers here contribute to decentralized energy grids, battery storage innovation, and strategies to phase out fossil fuels.
- Climate Science: Investigates the Earth’s climate system, analyzing variables like greenhouse gas emissions, sea-level rise, atmospheric circulation, and cryosphere dynamics. Climate scientists build predictive models to forecast temperature change, precipitation variability, and extreme weather patterns. This knowledge informs global accords like the Paris Agreement and supports adaptive infrastructure planning.
- Applications:
- Development of renewable energy technologies: Environmental science informs the design, deployment, and scaling of wind farms, solar energy systems, tidal power facilities, and bioenergy. It also provides environmental impact assessments (EIAs) to ensure sustainable energy transitions that minimize harm to ecosystems and communities.
- Waste management and recycling: This includes both technological and behavioral approaches. Environmental scientists assess municipal solid waste flows, promote composting and biodegradable materials, and design circular economy systems to reduce landfill dependence. Innovations such as anaerobic digestion, pyrolysis, and e-waste recovery have transformed how waste is managed in modern societies.
- Sustainable policy development: Experts in this field shape environmental regulations and governance frameworks at local, national, and international levels. They contribute to environmental impact statements, emissions trading systems, conservation laws, and water-use regulations. Key tools include environmental economics, ecosystem valuation, and environmental justice frameworks.
- Biodiversity conservation: By integrating population ecology, genetics, and geospatial analysis, environmental science contributes to designing conservation strategies that protect endangered species and critical habitats. Technologies such as remote sensing and DNA barcoding enhance monitoring capabilities and policy enforcement.
- Urban sustainability and green infrastructure: Environmental science supports the creation of sustainable cities by informing green roof designs, permeable pavements, urban forestry, and smart water systems. It also influences urban planning by promoting walkability, energy efficiency, and resilience to climate risks.
- Global monitoring networks: Environmental scientists contribute to satellite-based platforms, biosphere observatories, and real-time sensor networks that track changes in air quality, deforestation, water pollution, and biodiversity loss. For instance, platforms such as the NASA EarthData system provide open-access data for climate and environmental research worldwide.
- Public engagement and environmental literacy: Through citizen science, environmental education, and advocacy, professionals raise awareness of sustainability challenges and promote behavior change. This empowers communities to participate in conservation and demand responsible environmental governance.
Environmental science is pivotal to solving today’s most pressing challenges—from climate change and habitat destruction to resource scarcity and pollution. As the world transitions toward green technologies, low-carbon economies, and sustainable development pathways, the role of environmental scientists continues to grow in both significance and scope.
Importance of Science in the Modern World
- Advancing Knowledge and Expanding Human Understanding:
- Scientific inquiry is the cornerstone of modern civilization, shaping how we understand and interact with the world around us. It has transformed humanity’s relationship with nature through systematic observation, experimentation, and analysis. Across disciplines—physics, chemistry, biology, earth sciences, and beyond—scientific discovery has deepened our insights into natural laws, life processes, and cosmic phenomena. From Newton’s laws of motion to the quantum realm of Planck and Schrödinger, science has charted a course from the macro to the micro, revealing the complex mechanisms that govern both the universe and human biology.
- Major milestones like the discovery of DNA’s double-helix structure have revolutionized medicine, agriculture, and biotechnology. Similarly, developments in particle physics, such as the Higgs boson discovery at CERN, confirmed theoretical elements of the Standard Model and advanced our understanding of how particles acquire mass. These achievements illustrate science’s role in validating hypotheses and uncovering the building blocks of reality.
- In astronomy and space science, instruments like the Hubble Space Telescope and the James Webb Space Telescope allow scientists to observe light from galaxies formed just hundreds of millions of years after the Big Bang. These observations are not only expanding the frontiers of astrophysics but also answering fundamental questions about the origin and fate of the universe. Spectroscopic analysis of distant atmospheres may soon allow us to detect signs of extraterrestrial life or Earth-like planets.
- Scientific research also provides critical infrastructure for the advancement of other knowledge domains. For example, breakthroughs in materials science have yielded nanomaterials, quantum dots, and superconductors that are vital to energy storage, medical diagnostics, and electronics. Environmental science enables climate modeling and conservation efforts that support global sustainability goals. Behavioral science, when informed by empirical research, leads to more effective education systems, public health campaigns, and policy decisions.
- Moreover, science informs innovation. Technologies that are now integral to daily life—including smartphones, GPS, vaccines, and magnetic resonance imaging (MRI)—originated in laboratories and research centers. Science underpins our economic development by driving industrial competitiveness, automating production, and creating entirely new sectors such as biotechnology, space commerce, and quantum computing.
- A scientifically literate public is better positioned to navigate complex societal issues such as pandemics, climate change, food security, and ethical challenges in genetics and AI. Science education fosters habits of critical thinking, skepticism, and evidence-based reasoning, all of which are essential in countering misinformation and making responsible choices. In democratic societies, this ensures more rational policy debates and informed civic participation.
- The cultural and philosophical implications of science are equally profound. It reshapes our place in the universe, from Copernicus’ heliocentric model to the modern understanding that human life is a result of billions of years of cosmic evolution. Science also strengthens the bridge between cultures by providing a universal language of inquiry and a shared endeavor across national and ideological boundaries.
- Global scientific cooperation has also produced powerful collaborative institutions such as the International Thermonuclear Experimental Reactor (ITER), designed to advance nuclear fusion, and the Intergovernmental Panel on Climate Change (IPCC), which informs climate policies worldwide. A notable digital resource, ScienceDirect, offers access to cutting-edge peer-reviewed research and is instrumental in disseminating scientific knowledge globally.
- As society becomes more reliant on technology and data, the ethical responsibility of science grows. Scientists now play a pivotal role in guiding responsible innovation, preventing the misuse of knowledge, and ensuring that technological progress aligns with societal values and environmental sustainability.
- Ultimately, science is not merely a body of knowledge, but a transformative force that enriches lives, enables progress, and deepens our connection to the universe. It is the foundation of problem-solving, innovation, and resilience in an ever-changing world.
- Improving Lives through Innovation and Technology:
- The influence of scientific discovery on everyday life is immense and far-reaching. In virtually every domain—from health care and agriculture to energy and transportation—science has empowered transformative innovations that improve the quality, longevity, and sustainability of human life. One of the most groundbreaking advancements in recent years is the development of mRNA vaccines, which played a pivotal role in mitigating the COVID-19 pandemic. These vaccines not only curbed the spread of a global health crisis but also opened new frontiers in immunization strategies against diseases like malaria, influenza, and certain cancers.
- The fusion of science and engineering has yielded life-saving diagnostic tools such as MRI and CT scanners, which revolutionized medical imaging and non-invasive diagnosis. Similarly, advancements in computer science and materials engineering have led to the creation of supercomputers, high-capacity batteries, and wearable health monitors. These innovations reflect a cumulative legacy of basic scientific research translated into impactful real-world solutions.
- In the realm of energy, science-driven breakthroughs in photovoltaics, wind turbines, and grid-scale storage technologies have made renewable energy more efficient and economically viable. Solar panels, for example, owe their high efficiency to innovations in semiconductors and nanostructured materials—products of solid-state physics and materials chemistry. Smart grids, powered by AI and predictive analytics, optimize energy distribution and reduce environmental impact.
- Transportation has been similarly transformed by scientific innovation. Electric vehicles, hybrid propulsion systems, autonomous driving algorithms, and high-speed rail networks stem from multidisciplinary advances in mechanics, artificial intelligence, and control systems engineering. These technologies contribute to sustainable urban mobility and reduce our dependence on fossil fuels.
- In agriculture, genetically engineered crops and precision farming techniques are boosting food production while minimizing resource usage. Satellite imaging, drones, and soil sensors allow farmers to monitor crop health, optimize irrigation, and reduce pesticide use. Organizations like CGIAR foster global collaboration in agricultural science to build climate-resilient farming systems and combat food insecurity in vulnerable regions.
- The digital revolution—driven by physics, electronics, and software engineering—has transformed how people connect, work, and learn. The internet, smartphones, cloud computing, and machine learning are daily tools made possible by decades of scientific exploration into signal processing, algorithm design, and semiconductor technology.
- Looking ahead, continued investment in scientific research and development (R&D) is crucial for anticipating and solving the challenges of the future. According to the U.S. National Science Foundation, the scientific enterprise not only underpins national innovation systems but also supports millions of high-skilled jobs and drives economic competitiveness across sectors.
- Addressing Global Challenges and Shaping the Future:
- In the face of urgent global challenges—such as pandemics, climate change, resource scarcity, and environmental degradation—science is an indispensable tool for understanding, managing, and resolving crises. Evidence-based research allows policymakers, industries, and citizens to make informed decisions that are grounded in rigor and reproducibility. The work of the Intergovernmental Panel on Climate Change (IPCC) exemplifies how science can shape global governance by providing authoritative assessments of climate data and projections that guide international climate action.
- Scientific monitoring and modeling of Earth’s systems have revealed the dangers posed by anthropogenic activities, including greenhouse gas emissions, deforestation, ocean acidification, and biodiversity loss. Solutions like carbon capture technologies, reforestation strategies, and urban sustainability plans rely on cross-disciplinary knowledge from climatology, engineering, and economics. Environmental impact assessments and life-cycle analyses are now standard practice in both public infrastructure projects and corporate sustainability initiatives.
- Public health has become increasingly reliant on the convergence of disciplines such as genomics, epidemiology, and microbiology. From sequencing viral genomes to designing global vaccination strategies, science informs every aspect of health system preparedness. The World Health Organization (WHO) utilizes scientific findings to develop global health guidelines and orchestrate coordinated responses to pandemics and epidemics.
- The ability to anticipate and respond to natural disasters has been greatly enhanced through geoscientific research and technological innovation. Agencies like the U.S. Geological Survey (USGS) and the National Oceanic and Atmospheric Administration (NOAA) use seismology, meteorology, and satellite imaging to forecast and track earthquakes, hurricanes, floods, and wildfires. These capabilities save lives, guide urban planning, and bolster emergency response systems.
- Beyond technology and data, the ethical implications of scientific progress must be addressed. Fields like science communication, environmental ethics, and public policy ensure that advances are equitable, inclusive, and environmentally responsible. Transparent communication builds trust, empowers communities, and prevents the misuse of scientific knowledge. Public engagement platforms and publications such as Nature and Scientific American help bridge the gap between researchers and the general public, encouraging democratic dialogue and scientific literacy.
- In conclusion, science not only provides tools to solve complex problems but also equips society with the mindset and methodologies required to anticipate future issues, assess risks, and pursue holistic solutions. By embedding scientific principles into education, governance, and industry, humanity can shape a future that is more resilient, inclusive, and sustainable.
Why Study Science
Understanding the World
Science helps us make sense of the world we live in. It provides structured, evidence-based explanations for natural and human-made phenomena—from how cells divide to why the seasons change. By studying science, students learn to ask questions, seek answers through observation and experimentation, and draw conclusions supported by data. Science fuels curiosity and empowers us to understand everything from our own bodies to the complex systems that shape the environment and the universe.
Driving Technological and Social Progress
Scientific knowledge forms the foundation of nearly all modern technologies and innovations. Advances in medicine, clean energy, transportation, agriculture, and digital communication are rooted in scientific discovery. Whether it’s the development of vaccines, climate modeling, or artificial intelligence, science continues to shape the tools and solutions that improve our lives. A society grounded in scientific literacy is better equipped to make informed decisions and respond to global challenges like pandemics, food security, and climate change.
Developing Analytical and Problem-Solving Skills
Science education emphasizes evidence-based reasoning, logical thinking, and the application of knowledge to solve practical problems. Students learn to formulate hypotheses, design experiments, interpret data, and evaluate competing explanations—skills that are essential across academic disciplines and professional fields. These critical thinking abilities are transferable and highly valued in careers such as engineering, healthcare, law, data science, public policy, and business.
Preparing for University and Beyond
Studying science helps students build the intellectual discipline and academic habits required for success in university and lifelong learning. Science encourages precision, perseverance, and curiosity—traits that are invaluable in research and higher education. It also provides a strong foundation in mathematics, analytical writing, and technical communication. Students who study science are better prepared to adapt to complex, interdisciplinary fields and contribute meaningfully to the rapidly changing world of the 21st century.
🎥 Related Video – Why Study Emerging Technologies
Scientific discovery is the foundation upon which emerging technologies are built. From physics and chemistry to biology and environmental science, a strong scientific background helps students understand how innovation happens—and how to evaluate its impact.
This video from our Why Study series outlines eight compelling reasons to explore emerging technologies. It highlights how science and technology work together to solve real-world problems, drive innovation, and prepare students for a future shaped by both knowledge and curiosity.
Science: Conclusion
Science stands as a fundamental pillar of modern civilization, underpinning the knowledge systems and technologies that shape our lives. It is not merely a body of facts, but a methodical pursuit of truth through observation, experimentation, and logical reasoning. From the tiniest particles studied in physics to the vast ecosystems explored in environmental science, the scientific enterprise continues to expand the boundaries of human understanding.
Scientific discovery fuels technological innovation, social progress, and economic growth. It empowers us to combat diseases, improve agriculture, create clean energy, and even explore outer space. Equally important, it fosters critical thinking, skepticism, and informed decision-making—traits that are essential for democratic societies and individual empowerment. As we face complex global challenges such as climate change, pandemics, and resource depletion, science remains our most reliable tool for generating sustainable solutions.
The collaborative and interdisciplinary nature of science has never been more crucial. Breakthroughs increasingly emerge at the intersection of disciplines—from bioengineering to quantum computing—requiring integrative thinking and global cooperation. Initiatives like the Horizon Europe framework exemplify how governments and institutions worldwide are investing in research to drive inclusive innovation and resilience.
In education, science equips students with the tools to ask meaningful questions and seek evidence-based answers, nurturing curiosity and lifelong learning. For aspiring scientists, engineers, and informed citizens alike, the study of science is not just about knowledge—it’s about building a better future for all.
Science: Review Questions with Detailed Answers
Q1. Explain Newton’s Second Law of Motion and provide a real-world example of its application.
Answer:
- Newton’s Second Law of Motion states that the acceleration of an object is directly proportional to the net force acting upon it and inversely proportional to its mass. Mathematically, it is expressed as:
where
- Real-World Example:
Consider pushing a shopping cart. If you apply a constant force, the acceleration of the cart depends on its mass. An empty cart (lower mass) accelerates more quickly than a fully loaded cart (higher mass) when the same force is applied. This demonstrates the inverse relationship between mass and acceleration in Newton’s Second Law.
Q2. Describe the process of photosynthesis, including the main reactants and products.
Answer:
Photosynthesis is the biological process by which green plants, algae, and some bacteria convert light energy into chemical energy stored in glucose. It occurs primarily in the chloroplasts of plant cells.
Main Reactants:
- Carbon Dioxide: Taken in from the atmosphere through stomata
- Water : Absorbed by plant roots from the soil.
- Light Energy: Captured from sunlight by chlorophyll.
Main Products:
- Glucose
- Oxygen Released into the atmosphere as a byproduct.
Overall Equation:
Q3. What is the difference between an ionic bond and a covalent bond? Provide examples of each.
Answer:
Ionic Bond:
- Definition: Formed when one atom transfers one or more electrons to another atom, resulting in the formation of oppositely charged ions that attract each other.
- Characteristics: Typically occurs between metals and non-metals. High melting and boiling points. Conducts electricity when molten or dissolved in water.
- Example:
Sodium chloride (NaCl)- sodium (Na) donates an electron to chlorine (Cl), forming NA+ and Cl– ions.
Covalent Bond:
- Definition: Formed when two atoms share one or more pairs of electrons to achieve a stable electron configuration.
- Characteristics: Typically occurs between non-metals. Can form molecules with low melting and boiling points. Poor conductors of electricity.
- Example: – each hydrogen atom shares an electron with the oxygen atom, forming covalent bonds.
Q4. Explain the concept of energy conservation and its significance in physical sciences.
Answer:
Energy Conservation is a fundamental principle stating that energy cannot be created or destroyed in an isolated system; it can only be transformed from one form to another. The total energy within a closed system remains constant over time.
Significance in Physical Sciences:
- Predictive Power: Allows scientists to predict the behavior of physical systems by accounting for all energy transformations.
- Problem-Solving: Essential for solving problems in mechanics, thermodynamics, electromagnetism, and other fields by ensuring energy balances are maintained.
- Understanding Processes: Helps in understanding natural phenomena, such as how energy flows in ecosystems or how machines operate efficiently.
- Engineering Applications: Critical in designing energy-efficient systems, renewable energy technologies, and managing energy resources effectively.
Q5. Define the term “ecosystem” and describe its main components.
Answer:
An Ecosystem is a biological community of interacting organisms and their physical environment, functioning as a system. It encompasses all living (biotic) and non-living (abiotic) components in a particular area and the interactions between them.
Main Components:
- Biotic Components:
- Producers (Autotrophs): Organisms like plants and algae that produce their own food through photosynthesis.
- Consumers (Heterotrophs): Organisms that consume other organisms for energy, including herbivores, carnivores, and omnivores.
- Decomposers: Organisms like bacteria and fungi that break down dead matter, recycling nutrients back into the ecosystem.
- Abiotic Components:
- Sunlight: Provides the primary energy source for most ecosystems.
- Water: Essential for all living organisms and involved in various biochemical processes.
- Minerals and Nutrients: Necessary for the growth and development of organisms.
- Temperature: Influences the metabolic rates and distribution of organisms.
- Atmospheric Gases: Such as oxygen and carbon dioxide, which are vital for respiration and photosynthesis.
Interactions:
Energy flows through ecosystems via food webs, and matter cycles through biogeochemical cycles, ensuring the sustainability and balance of the ecosystem.
Q6. What is the law of universal gravitation and how does it apply to celestial bodies?
Answer:
Law of Universal Gravitation, formulated by Sir Isaac Newton, states that every point mass attracts every other point mass in the universe with a force that is directly proportional to the product of their masses and inversely proportional to the square of the distance between their centers. The mathematical expression is:
where: F is the gravitational force, G is the gravitational constant, m1 and m2 are the masses of the two objects. r is the distance between the centers of the two masses.
Application to Celestial Bodies:
- Orbital Motion: Governs the motion of planets around the Sun, moons around planets, and satellites around celestial bodies.
- Formation of Celestial Structures: Responsible for the aggregation of matter in stars, planets, and galaxies.
- Tides: The gravitational pull of the Moon and the Sun causes tides on Earth.
- Stability of the Solar System: Ensures that celestial bodies maintain stable orbits without spiraling into each other or flying apart.
Q7. Describe the difference between kinetic and potential energy, providing examples of each.
Answer:
- Kinetic Energy (KE):
- Definition: The energy an object possesses due to its motion.
- Formula:
- Examples:
- A moving car has kinetic energy proportional to its mass and the square of its velocity.
- A flowing river possesses kinetic energy due to the movement of water.
- Potential Energy (PE):
- Definition: The energy stored in an object due to its position, condition, or configuration.
- Formula:
(for gravitational potential energy near Earth’s surface)
- Examples:
- A book held at a height above the ground has gravitational potential energy.
- A compressed spring stores elastic potential energy.
Key Difference:
Kinetic energy is associated with motion, while potential energy is associated with position or configuration. The total mechanical energy of a system is the sum of its kinetic and potential energies.
Q8. What is the pH scale, and how does it relate to acidity and basicity?
Answer:
The pH Scale is a logarithmic scale used to measure the acidity or basicity (alkalinity) of an aqueous solution. It ranges from 0 to 14, with 7 being neutral.
- pH < 7: Indicates an acidic solution. The lower the pH, the higher the acidity and the higher the concentration of hydrogen ions (H+)
- pH = 7: Indicates a neutral solution, such as pure water, where the concentration of hydrogen ions equals that of hydroxide ions (OH–)
- pH > 7: Indicates a basic or alkaline solution. The higher the pH, the greater the basicity and the higher the concentration of hydroxide ions.
Relation to Acidity and Basicity:
- Acidic Solutions: High concentration of H+ ions.
- Basic Solutions: Low concentration of H+ ions and a high concentration of OH– ions.
Applications:
The pH scale is crucial in chemistry, biology, environmental science, medicine, and various industries to monitor and control the acidity or basicity of solutions for desired reactions and processes.
Q9. Explain the concept of genetic inheritance using Mendel’s laws.
Answer:
Genetic Inheritance refers to the transmission of traits from parents to offspring through genes. Gregor Mendel, the father of genetics, established fundamental principles that describe how traits are inherited.
Mendel’s Laws:
- Law of Segregation:
- Statement: Each individual possesses two alleles for each gene, which segregate (separate) during the formation of gametes (eggs and sperm), so that each gamete carries only one allele for each gene.
- Implication: Offspring inherit one allele from each parent, restoring the pair.
- Law of Independent Assortment:
- Statement: Genes for different traits assort independently of one another during gamete formation.
- Implication: The inheritance of one trait generally does not affect the inheritance of another, leading to genetic variation.
Examples:
- Monohybrid Cross: Studying a single trait, such as flower color in pea plants, demonstrates the Law of Segregation.
- Dihybrid Cross: Studying two traits simultaneously, like seed shape and color, illustrates the Law of Independent Assortment.
Significance:
Mendel’s laws form the foundation of classical genetics, enabling the prediction of trait inheritance patterns, understanding genetic diversity, and informing fields like agriculture, medicine, and evolutionary biology.
Q10. What are the three states of matter, and how do they differ in terms of particle arrangement and energy?
Answer:
The Three States of Matter are:
- Solid:
- Particle Arrangement: Particles are closely packed in a fixed, orderly pattern.
- Energy: Particles have low kinetic energy, primarily vibrating in place.
- Characteristics: Definite shape and volume, incompressible, rigid structure.
- Liquid:
- Particle Arrangement: Particles are close together but not in a fixed position, allowing them to flow past one another.
- Energy: Particles have higher kinetic energy than in solids, enabling movement while maintaining proximity.
- Characteristics: Indefinite shape (takes the container’s shape) but definite volume, slightly compressible, fluidity.
- Gas:
- Particle Arrangement: Particles are widely spaced and move freely in all directions.
- Energy: Particles possess high kinetic energy, overcoming intermolecular forces.
- Characteristics: Indefinite shape and volume, highly compressible, expand to fill any container.
Differences in Particle Arrangement and Energy:
- Arrangement: Solids have a fixed, orderly structure; liquids have a disordered but close arrangement; gases have a highly disordered and widely spaced arrangement.
- Energy: As matter transitions from solid to liquid to gas, the kinetic energy of particles increases, allowing greater freedom of movement and overcoming intermolecular forces.
Additional State – Plasma: While not one of the traditional three, plasma is often considered the fourth state of matter, consisting of ionized particles with high energy, found in stars and fluorescent lights.
Q11. Define the term “electromagnetic spectrum” and list its main regions in order of increasing frequency.
Answer:
The Electromagnetic Spectrum is the range of all types of electromagnetic radiation, which are waves of electric and magnetic fields propagating through space. These waves vary in wavelength and frequency but travel at the same speed in a vacuum (approximately 3 x 108 m/s).
Main Regions of the Electromagnetic Spectrum (Ordered by Increasing Frequency):
- Radio Waves:
- Wavelength: > 1 meter
- Frequency: < 3 x 109 Hz
- Uses: Broadcasting, communication, radar.
- Microwaves:
- Wavelength: 1 meter to 1 millimeter
- Frequency:
- 3 x 109 Hz to 3 x 1012 Hz
- Uses: Cooking, satellite communication, wireless networking.
- Infrared (IR) Radiation:
- Wavelength: 1 millimeter to 700 nanometers
- Frequency:
- 3 x 1012 Hz to 3 x 1014 Hz
- Uses: Thermal imaging, remote controls, heating.
- Visible Light:
- Wavelength: 700 nanometers to 400 nanometers
- Frequency:
- 4.3 x 1014 Hz to 7.5 x 1014 Hz
- Uses: Human vision, lighting, photography.
- Ultraviolet (UV) Radiation:
- Wavelength: 400 nanometers to 10 nanometers
- Frequency:
- 7.5 x 1014 Hz to 3 x 1016 Hz
- Uses: Sterilization, fluorescent lighting, medical imaging.
- X-Rays:
- Wavelength: 10 nanometers to 0.01 nanometers
- Frequency:
- 3 x 1016 Hz to 3 x 1019 Hz
- Uses: Medical imaging, security screening, material analysis.
- Gamma Rays:
- Wavelength: < 0.01 nanometers
- Frequency: >
- Uses: Cancer treatment, nuclear reactions, astrophysics.
- Significance:
Each region of the electromagnetic spectrum has unique properties and applications, ranging from everyday technologies like radio and microwaves to specialized fields such as medical imaging and astrophysics.Q12. What is the principle of conservation of mass, and how does it apply to chemical reactions?
Answer:
The Principle of Conservation of Mass states that mass cannot be created or destroyed in a closed system through ordinary chemical reactions or physical transformations. The total mass of reactants equals the total mass of products in any chemical reaction.Application to Chemical Reactions:
- Balanced Equations: Chemical equations must be balanced to reflect the conservation of mass. This involves ensuring that the number of atoms for each element is the same on both the reactant and product sides.Example:
Unbalanced:Balanced:
Here, 4 hydrogen atoms and 2 oxygen atoms are present on both sides, satisfying mass conservation.
- Stoichiometry: The principle allows chemists to calculate the amounts of reactants and products involved in reactions, enabling precise control and prediction of reaction outcomes.
- Industrial Processes: Ensures efficiency in manufacturing, where inputs and outputs are carefully measured to minimize waste and optimize resource use.
Significance:
Understanding and applying the conservation of mass is fundamental in chemistry, ensuring accurate experimentation, industrial production, environmental management, and theoretical modeling of chemical processes.These questions and answers cover a broad range of fundamental science topics, including physics, chemistry, biology, and environmental science. They are designed to reinforce key concepts and provide comprehensive understanding for students preparing for exams or seeking to deepen their knowledge in STEM disciplines.
- Balanced Equations: Chemical equations must be balanced to reflect the conservation of mass. This involves ensuring that the number of atoms for each element is the same on both the reactant and product sides.Example:
Science: Thought-Provoking Questions with Answers
1. How does the process of photosynthesis impact the global carbon cycle and climate regulation?
Answer: Photosynthesis plays a pivotal role in the global carbon cycle and climate regulation by converting carbon dioxide (
) from the atmosphere into organic compounds and oxygen. Here’s how it impacts these systems:
Carbon Sequestration: Plants, algae, and certain bacteria absorb
during photosynthesis to produce glucose (
) and other carbohydrates. This process effectively removes carbon from the atmosphere, helping to mitigate the greenhouse effect and regulate global temperatures.
Oxygen Production: Photosynthesis releases oxygen (
) as a byproduct, which is essential for the respiration of most living organisms and maintains the balance of atmospheric gases.
Ecosystem Support: By forming the base of the food web, photosynthesis supports a vast array of life forms, ensuring ecosystem stability and biodiversity.
Climate Regulation: The sequestration of carbon through photosynthesis helps offset anthropogenic
emissions from activities like fossil fuel combustion, thus playing a critical role in climate regulation and reducing the pace of global warming.
Long-Term Storage: Organic carbon can be stored in biomass, soils, and oceans, serving as a long-term reservoir that influences atmospheric
levels over geological timescales.
In summary, photosynthesis is fundamental to maintaining atmospheric balance, supporting life, and regulating the Earth’s climate by controlling
levels and producing oxygen.
2. Explain the significance of the periodic table in understanding chemical behavior and predicting reactions.
Answer: The periodic table is a fundamental tool in chemistry that organizes all known chemical elements in a systematic manner based on their atomic number, electron configurations, and recurring chemical properties. Its significance lies in several key areas:
Organization of Elements: Elements are arranged in order of increasing atomic number into rows (periods) and columns (groups or families) with similar properties. This arrangement highlights periodic trends such as electronegativity, ionization energy, and atomic radius.
Predicting Chemical Behavior: Elements within the same group often exhibit similar chemical behaviors because they have the same number of valence electrons. For instance, the noble gases are inert, while the alkali metals are highly reactive. This predictability aids chemists in anticipating how elements will react with each other.
Understanding Periodic Trends: The periodic table reveals trends that explain why elements behave the way they do. For example:
- Atomic Radius: Generally decreases across a period and increases down a group.
- Ionization Energy: Tends to increase across a period and decrease down a group.
- Electronegativity: Increases across a period and decreases down a group.
Facilitating Discovery of New Elements: The periodic table guides scientists in discovering new elements by predicting their properties and placement based on existing trends.
Simplifying Chemical Nomenclature and Classification: It provides a standardized framework for naming and classifying elements and compounds, making communication in the scientific community more efficient.
Applications in Various Fields: Beyond pure chemistry, the periodic table is essential in materials science, biology, environmental science, and engineering for designing compounds, understanding biochemical processes, and developing new technologies.
In essence, the periodic table is invaluable for organizing chemical knowledge, predicting element behavior, and facilitating advancements across multiple scientific disciplines.
3. Describe the structure of DNA and explain how it encodes genetic information.
Answer: Deoxyribonucleic Acid (DNA) is the hereditary material in almost all living organisms and many viruses. Its structure is fundamental to its function in encoding genetic information. Here’s a detailed description:
Double Helix Structure: DNA consists of two long strands that coil around each other to form a double helix, resembling a twisted ladder. This structure was first described by James Watson and Francis Crick in 1953.
Nucleotides: Each strand is composed of repeating units called nucleotides. Each nucleotide consists of three components:
- Phosphate Group: Attached to the 5′ carbon of the sugar, forming the backbone of the DNA strand.
- Deoxyribose Sugar: A five-carbon sugar that connects the phosphate group to the nitrogenous base.
- Nitrogenous Base: There are four types—adenine (A), thymine (T), cytosine (C), and guanine (G).
Base Pairing: The two DNA strands are complementary, with bases pairing specifically:
- Adenine (A) pairs with Thymine (T) via two hydrogen bonds.
- Cytosine (C) pairs with Guanine (G) via three hydrogen bonds. This base-pairing rule ensures accurate replication of genetic information.
Antiparallel Orientation: The two strands run in opposite directions—one from the 5′ to 3′ end and the other from 3′ to 5′ end. This antiparallel arrangement is crucial for replication and transcription processes.
Genetic Encoding:
- Genes: Segments of DNA that contain instructions for building proteins. Each gene is a sequence of bases that specifies the order of amino acids in a protein.
- Codons: Three-base sequences on the DNA (and corresponding mRNA) that code for specific amino acids.
- Regulatory Regions: Non-coding sequences that control the expression of genes, determining when and where proteins are produced.
Replication: DNA’s double helix unwinds, and each strand serves as a template for the formation of a new complementary strand, ensuring genetic information is passed accurately to new cells.
Mutation and Variation: Changes in the DNA sequence (mutations) can lead to variations in genetic information, which are essential for evolution but can also cause genetic disorders.
In summary, DNA’s double helix structure with specific base-pairing rules allows it to store and transmit genetic information reliably, serving as the blueprint for an organism’s development, functioning, and reproduction.
4. What is the law of conservation of energy, and how does it apply to biological systems?
Answer: The Law of Conservation of Energy is a fundamental principle in physics stating that energy cannot be created or destroyed in an isolated system; it can only be transformed from one form to another or transferred between systems. The total energy within a closed system remains constant over time.
Application to Biological Systems:
Energy Transformation: Biological organisms constantly transform energy from one form to another to sustain life processes.
- Photosynthesis: Plants convert light energy into chemical energy stored in glucose molecules.
- Cellular Respiration: Cells break down glucose to release stored chemical energy for various functions, producing
Energy Flow: Energy flows through biological systems in a unidirectional manner—from producers (like plants) to consumers (herbivores, carnivores) and decomposers (fungi, bacteria). At each step, energy is transformed, often with some loss as heat due to inefficiencies, aligning with the second law of thermodynamics.
Metabolic Processes: All metabolic activities, including muscle contraction, nerve impulse transmission, and biosynthesis of molecules, involve energy transformations adhering to conservation principles.
Ecosystem Dynamics: In ecosystems, energy captured by photosynthesis moves through food webs via trophic levels. The total energy input equals the energy output plus the energy stored, ensuring energy conservation within the ecosystem.
Homeostasis: Organisms maintain internal stability by regulating energy intake, storage, and expenditure. For example, humans balance caloric intake with metabolic needs and physical activity.
Biochemical Reactions: Enzymatic reactions in cells involve the transformation of energy to drive endergonic (energy-requiring) processes, ensuring that energy is conserved and utilized efficiently.
In essence, the law of conservation of energy underpins all biological processes, ensuring that energy is systematically managed and transformed to support life, maintain ecological balance, and drive the intricate functions within living organisms.
5. How do enzymes function as biological catalysts, and why are they essential for metabolic reactions?
Answer: Enzymes are proteins that act as biological catalysts, accelerating chemical reactions without being consumed in the process. They are essential for facilitating and regulating the vast array of metabolic reactions necessary for life.
Functioning as Biological Catalysts:
Lowering Activation Energy: Enzymes reduce the activation energy required for a reaction to occur, allowing reactions to proceed faster and under milder conditions than would otherwise be possible.
Active Site: Each enzyme has a specific region called the active site where substrates (reactant molecules) bind. The active site’s shape and chemical environment stabilize the transition state, facilitating the reaction.
Substrate Specificity: Enzymes exhibit high specificity, meaning each enzyme typically catalyzes a particular reaction or set of closely related reactions. This specificity is due to the precise fit between the enzyme’s active site and its substrate, often described by the “lock and key” or “induced fit” models.
Reusability: Since enzymes are not consumed in the reactions they catalyze, they can be used repeatedly to facilitate multiple reaction cycles.
Regulation: Enzyme activity can be regulated by various factors, including temperature, pH, and the presence of inhibitors or activators. This regulation allows cells to control metabolic pathways efficiently.
Essentiality for Metabolic Reactions:
Efficiency: Metabolic reactions occur at rates necessary to sustain life. Enzymes ensure that these reactions proceed swiftly enough to meet cellular demands.
Energy Management: By lowering activation energies, enzymes enable cells to carry out reactions without requiring excessive energy input, conserving energy for other vital processes.
Pathway Integration: Enzymes facilitate complex metabolic pathways by linking multiple reactions in a coordinated sequence, ensuring that intermediates are efficiently converted to final products.
Homeostasis: Enzymes help maintain internal balance by regulating the rates of biochemical reactions in response to the cell’s needs, allowing organisms to adapt to changing environments.
Synthesis and Degradation: Enzymes are involved in both the synthesis of essential molecules (anabolic pathways) and the breakdown of compounds for energy (catabolic pathways), making them central to cellular function and survival.
In summary, enzymes are indispensable for life as they catalyze and regulate the myriad chemical reactions that underpin biological processes, ensuring that cells operate efficiently, respond to environmental changes, and maintain structural and functional integrity.
6. What are the primary differences between prokaryotic and eukaryotic cells, and how do these differences influence their respective functions?
Answer: Prokaryotic and eukaryotic cells represent the two fundamental types of cellular organization in living organisms. They differ in structure, complexity, and functional capabilities, which influence their roles in nature.
Primary Differences:
Nuclear Region:
- Prokaryotic Cells:
- Lack a true nucleus.
- DNA is located in a region called the nucleoid, which is not membrane-bound.
- Eukaryotic Cells:
- Possess a true nucleus enclosed by a nuclear membrane.
- DNA is organized into multiple linear chromosomes within the nucleus.
- Prokaryotic Cells:
Cell Size:
- Prokaryotic Cells:
- Generally smaller (1-10 micrometers in diameter).
- Eukaryotic Cells:
- Larger (10-100 micrometers in diameter).
- Prokaryotic Cells:
Organelles:
- Prokaryotic Cells:
- Lack membrane-bound organelles.
- May contain specialized structures like ribosomes, flagella, and pili.
- Eukaryotic Cells:
- Contain various membrane-bound organelles (e.g., mitochondria, endoplasmic reticulum, Golgi apparatus, lysosomes, chloroplasts in plants).
- Have a complex internal structure facilitating compartmentalization of functions.
- Prokaryotic Cells:
Genetic Material:
- Prokaryotic Cells:
- Typically have a single, circular chromosome.
- May contain plasmids—small, circular DNA molecules separate from chromosomal DNA.
- Eukaryotic Cells:
- Multiple, linear chromosomes housed within the nucleus.
- DNA associated with histone proteins, forming chromatin.
- Prokaryotic Cells:
Reproduction:
- Prokaryotic Cells:
- Reproduce asexually through binary fission—a simple division process.
- Eukaryotic Cells:
- Reproduce both asexually (mitosis) and sexually (meiosis), involving complex division processes.
- Prokaryotic Cells:
Cell Wall Composition:
- Prokaryotic Cells:
- Often have a rigid cell wall composed of peptidoglycan (in bacteria).
- Eukaryotic Cells:
- Plant cells have cell walls made of cellulose; fungi have walls made of chitin.
- Animal cells lack a cell wall.
- Prokaryotic Cells:
Ribosomes:
- Prokaryotic Cells:
- Have smaller ribosomes (70S).
- Eukaryotic Cells:
- Possess larger ribosomes (80S) found in the cytoplasm and on the rough endoplasmic reticulum.
- Prokaryotic Cells:
Influence on Functions:
Metabolic Diversity: Prokaryotes, especially bacteria, exhibit immense metabolic diversity, allowing them to inhabit various environments and perform roles like nitrogen fixation, decomposition, and pathogenesis. Eukaryotes, with their compartmentalized organelles, can perform complex processes such as cellular respiration in mitochondria and photosynthesis in chloroplasts.
Genetic Regulation: Eukaryotic cells have more intricate mechanisms for gene regulation, enabling specialization and multicellularity. Prokaryotic cells, while capable of genetic regulation and horizontal gene transfer, generally operate as unicellular organisms.
Response to Environment: The simplicity and efficiency of prokaryotic cells allow rapid responses to environmental changes, such as antibiotic resistance development. Eukaryotic cells, with their complex structures, are better suited for maintaining internal stability in multicellular organisms.
Reproduction and Evolution: Eukaryotes’ sexual reproduction introduces genetic variation, driving evolution and complexity. Prokaryotes’ asexual reproduction allows for quick population growth and adaptation through mutations and gene transfer.
In summary, the structural and functional distinctions between prokaryotic and eukaryotic cells underpin their respective roles in ecosystems, their adaptability, and the complexity of the organisms they constitute.
7. How does the process of natural selection drive evolution, and what evidence supports this mechanism?
Answer: Natural Selection is a fundamental mechanism of evolution proposed by Charles Darwin. It explains how species adapt and evolve over time through differential survival and reproduction of individuals with advantageous traits.
Process of Natural Selection:
Variation: Within a population, individuals exhibit variations in traits (e.g., coloration, size, speed) due to genetic differences and mutations.
Inheritance: Some of these traits are heritable, passed from parents to offspring through genetic material.
Differential Survival and Reproduction: In a given environment, individuals with traits better suited to their surroundings are more likely to survive and reproduce, passing those advantageous traits to the next generation.
Accumulation of Favorable Traits: Over successive generations, favorable traits become more common in the population, leading to adaptation to the environment.
Evidence Supporting Natural Selection:
Fossil Record:
- Transitional Forms: Fossils show intermediary stages between major groups of organisms, indicating gradual evolutionary changes (e.g., Archaeopteryx linking dinosaurs and birds).
- Chronological Succession: The fossil record displays a timeline of life forms appearing in an order consistent with evolutionary predictions.
Biogeography:
- Geographical Distribution: Similar species are found in similar environments regardless of geographical separation, while unique species are often found in isolated regions (e.g., Darwin’s finches in the Galápagos Islands).
Comparative Anatomy:
- Homologous Structures: Structures with similar anatomy but different functions (e.g., the limb bones of humans, whales, and bats) suggest common ancestry.
- Vestigial Structures: Reduced or non-functional structures (e.g., human tailbone, whale pelvic bones) indicate evolutionary remnants.
Comparative Embryology:
- Similar Developmental Stages: Embryos of different species exhibit similar stages of development, reflecting common genetic heritage.
Molecular Biology:
- Genetic Similarities: DNA and protein sequences show higher similarity among closely related species, supporting common descent.
- Genomic Evidence: Shared genetic markers and gene sequences across species corroborate evolutionary relationships.
Observed Instances of Evolution:
- Antibiotic Resistance: Bacteria evolve resistance to antibiotics through natural selection, demonstrating rapid evolutionary changes.
- Peppered Moth Adaptation: The color variation in peppered moths in response to industrial pollution serves as a classic example of natural selection in action.
Artificial Selection:
- Selective Breeding: Humans have bred plants and animals for specific traits (e.g., dog breeds, crop varieties), showcasing how selection can shape species over generations.
Genetic Drift and Gene Flow:
- While distinct from natural selection, these processes interplay with natural selection to influence allele frequencies in populations, supporting the broader evolutionary framework.
In summary, natural selection drives evolution by favoring advantageous traits that enhance survival and reproduction, leading to the adaptation and diversification of species. The convergence of multiple lines of evidence from various scientific disciplines robustly supports this mechanism as a cornerstone of evolutionary theory.
8. What roles do acids and bases play in biochemical reactions, and how is pH regulation crucial for cellular function?
Answer: Acids and bases are essential in biochemical reactions, influencing enzyme activity, protein structure, and overall cellular homeostasis. Their roles and the regulation of pH are critical for maintaining proper cellular function.
Roles in Biochemical Reactions:
Enzyme Function:
- Optimal pH: Enzymes have specific pH ranges where they exhibit maximum activity. Deviations can alter the ionization of amino acids at the active site, affecting substrate binding and catalysis.
- Denaturation: Extreme pH levels can lead to the denaturation of enzymes, causing loss of structure and function.
Protein Structure:
- Charge Interactions: Acids and bases influence the ionization states of amino acid side chains, affecting hydrogen bonding and electrostatic interactions that maintain protein tertiary and quaternary structures.
- Folding and Stability: Proper pH levels ensure correct protein folding, which is essential for their biological activity.
Metabolic Pathways:
- Reaction Rates: Acidic and basic conditions can accelerate or decelerate reaction rates, impacting metabolic fluxes.
- Intermediate Stability: Some metabolic intermediates are sensitive to pH, influencing pathway efficiency and directionality.
Buffer Systems:
- pH Maintenance: Acids and bases are integral to buffer systems that resist changes in pH, ensuring that biochemical reactions occur under stable conditions.
Importance of pH Regulation:
Cellular Homeostasis:
- Enzyme Activity: Maintaining optimal pH ensures that enzymes function efficiently, supporting vital processes like glycolysis, the Krebs cycle, and oxidative phosphorylation.
- Ion Transport: pH influences the proton gradient across membranes, crucial for ATP synthesis in mitochondria and chloroplasts.
Electrolyte Balance:
- Ionization States: Proper pH maintains the ionization states of electrolytes (e.g., sodium, potassium, calcium), which are essential for nerve impulse transmission and muscle contraction.
Genetic Material Protection:
- DNA and RNA Stability: Stable pH prevents denaturation and maintains the integrity of genetic material, ensuring accurate replication and transcription.
Signal Transduction:
- Receptor Function: pH affects the binding of ligands to receptors, influencing cellular signaling pathways and responses to external stimuli.
Waste Removal:
- Buffering Metabolic Byproducts: pH regulation aids in the removal and neutralization of metabolic byproducts (e.g., lactic acid), preventing acidosis or alkalosis.
Mechanisms of pH Regulation:
- Buffer Systems: Bicarbonate, phosphate, and protein buffers absorb excess
- Respiratory Control: Regulation of carbon dioxide levels through breathing adjusts blood pH by shifting the bicarbonate buffer equilibrium.
- Renal Regulation: Kidneys excrete or retain
- Cellular Pumps and Transporters: Active transport mechanisms adjust intracellular pH by moving ions across membranes.
In conclusion, acids and bases are integral to biochemical reactions and cellular processes, with precise pH regulation being essential for maintaining the structural integrity and functional efficiency of cells. Disruptions in pH balance can lead to impaired enzyme activity, metabolic dysfunction, and overall cellular distress.
9. How do the principles of thermodynamics apply to energy transfer in ecosystems?
Answer: Thermodynamics governs the principles of energy transfer and transformation in all physical and biological systems, including ecosystems. Understanding these principles helps elucidate how energy flows through ecosystems, supporting life and maintaining ecological balance.
Application of Thermodynamic Principles:
First Law of Thermodynamics (Energy Conservation):
- Energy Flow: In ecosystems, energy enters primarily through sunlight, captured by producers (plants, algae) via photosynthesis. This energy is then transferred through trophic levels—from producers to consumers and decomposers—without being created or destroyed.
- Energy Transformation: As energy moves through the ecosystem, it transforms from light energy to chemical energy, and eventually to thermal energy (heat), which dissipates into the environment.
Second Law of Thermodynamics (Entropy Increase):
- Energy Quality Degradation: With each energy transfer, some energy becomes less available for doing work, typically lost as heat. This aligns with the concept of increasing entropy, as energy disperses more randomly.
- Efficiency Limits: Energy transfer between trophic levels is inefficient, with only about 10% of the energy being passed on from one level to the next. The rest is lost, limiting the number of trophic levels in an ecosystem.
Energy Pyramids:
- Structure: Visual representations like energy pyramids illustrate the decrease in available energy at each trophic level, reflecting thermodynamic constraints.
- Implications: This structure explains why apex predators are fewer in number and biomass compared to primary consumers and producers.
Metabolic Rates and Energy Use:
- Basal Metabolic Rate (BMR): Organisms require a constant energy input to maintain basic physiological functions, which is dictated by thermodynamic principles.
- Heat Production: Cellular respiration converts chemical energy into ATP and releases excess energy as heat, contributing to the thermal balance within ecosystems.
Energy Storage and Release:
- Biomass Accumulation: Producers store energy in organic compounds, serving as energy reservoirs that sustain consumers and decomposers.
- Decomposition: Decomposers break down dead organic matter, releasing energy and recycling nutrients, facilitating continuous energy flow.
Energy Sources and Sustainability:
- Primary Energy Source: Sunlight is the fundamental energy source driving ecosystems, enabling the flow and transformation of energy necessary for life.
- Renewable Energy Use: Ecosystems rely on renewable energy flows, ensuring sustainability as long as energy input (e.g., sunlight) remains consistent.
Implications for Ecosystem Dynamics:
- Biodiversity and Stability: Efficient energy transfer supports diverse species and complex interactions, contributing to ecosystem resilience.
- Human Impact: Disruptions to energy flows (e.g., deforestation, pollution) can destabilize ecosystems, highlighting the importance of sustainable energy management.
In summary, the principles of thermodynamics provide a framework for understanding how energy is captured, transformed, and distributed within ecosystems. These principles explain the efficiency limitations, energy hierarchy, and sustainability of ecological communities, emphasizing the interconnectedness of energy dynamics and biological processes.
10. What is genetic drift, and how does it differ from natural selection in driving evolutionary change?
Answer: Genetic Drift is a mechanism of evolution that involves random changes in allele frequencies within a population, leading to evolutionary change independent of natural selection. It primarily affects small populations and can result in the loss or fixation of alleles purely by chance.
Key Characteristics of Genetic Drift:
Randomness: Unlike natural selection, which is a non-random process driven by environmental pressures favoring certain traits, genetic drift operates randomly without regard to an organism’s fitness.
Population Size Influence:
- Small Populations: More susceptible to genetic drift because random events can significantly alter allele frequencies.
- Large Populations: Genetic drift has a minimal effect due to the law of large numbers, which averages out random fluctuations.
Bottleneck Effect: A drastic reduction in population size due to an event (e.g., natural disaster) can cause a loss of genetic diversity as only a small subset of alleles survive.
Founder Effect: When a new population is established by a small number of individuals from a larger population, the new population may have different allele frequencies, reflecting the genetic makeup of the founders rather than the original population.
Differences from Natural Selection:
Directionality:
- Natural Selection: Leads to directional changes in allele frequencies based on differential survival and reproduction of individuals with advantageous traits.
- Genetic Drift: Causes allele frequencies to fluctuate randomly, without any predictable direction.
Influence of Fitness:
- Natural Selection: Alleles that confer higher fitness increase in frequency.
- Genetic Drift: Alleles may increase or decrease in frequency regardless of their impact on fitness.
Role in Evolution:
- Natural Selection: Drives adaptive evolution, leading to traits that enhance survival and reproduction in specific environments.
- Genetic Drift: Can lead to the random loss of alleles, reducing genetic variation, and sometimes leading to fixation of neutral or even deleterious alleles.
Impact on Genetic Diversity:
- Natural Selection: Can either increase diversity by favoring multiple advantageous traits or decrease it by favoring a single optimal trait.
- Genetic Drift: Generally decreases genetic diversity by randomly removing alleles from the gene pool.
Examples:
Genetic Drift: In a small isolated island population, a natural disaster might randomly kill individuals with certain alleles, altering the population’s genetic structure without any selective pressure.
Natural Selection: In a predator-rich environment, prey with better camouflage may survive longer and reproduce more, increasing the frequency of camouflage-related alleles.
Combined Effects: Both genetic drift and natural selection can act simultaneously, especially in small populations, influencing the evolutionary trajectory in complex ways.
In summary, genetic drift is a stochastic process that causes random changes in allele frequencies, contrasting with the deterministic nature of natural selection. While natural selection leads to adaptive evolution based on environmental pressures, genetic drift introduces randomness into the evolutionary process, particularly impacting small populations and contributing to genetic diversity or homogeneity.
11. How does the greenhouse effect regulate Earth’s temperature, and what are the implications of its enhancement due to human activities?
Answer: The Greenhouse Effect is a natural process by which certain gases in Earth’s atmosphere trap heat, preventing it from escaping into space, thus regulating the planet’s temperature to sustain life.
Mechanism of the Greenhouse Effect:
Solar Radiation: The Sun emits energy that reaches Earth, primarily as visible light and ultraviolet (UV) radiation.
Absorption and Reflection: About 30% of this incoming solar radiation is reflected back into space by clouds, aerosols, and Earth’s surface (albedo effect). The remaining 70% is absorbed by the Earth’s surface and atmosphere, warming the planet.
Infrared Emission: Earth emits energy back into space as infrared (IR) radiation due to its temperature.
Greenhouse Gas Absorption: Greenhouse gases (GHGs) such as carbon dioxide (
), methane (
), water vapor (
), and nitrous oxide (
) absorb a portion of this outgoing IR radiation, trapping heat in the atmosphere.
Re-emission of Heat: GHGs re-emit the absorbed IR radiation in all directions, including back towards Earth, leading to further warming of the surface and lower atmosphere.
Regulation of Earth’s Temperature:
- Natural Balance: The greenhouse effect maintains Earth’s average surface temperature around 15°C (59°F), enabling liquid water and supporting diverse life forms. Without it, Earth’s average temperature would plummet to approximately -18°C (0°F), making it inhospitable.
Enhancement Due to Human Activities:
Increased GHG Emissions:
- Fossil Fuel Combustion: Burning coal, oil, and natural gas for energy and transportation releases significant amounts of
- Deforestation: Reduces the number of trees that can absorb
- Agriculture and Industry: Emit methane from livestock and nitrous oxide from fertilizers and industrial processes.
- Fossil Fuel Combustion: Burning coal, oil, and natural gas for energy and transportation releases significant amounts of
Consequences of Enhanced Greenhouse Effect:
Global Warming: Increased concentrations of GHGs intensify the greenhouse effect, leading to a rise in Earth’s average temperature.
Climate Change: Altered weather patterns, including more frequent and severe storms, droughts, heatwaves, and shifts in precipitation distribution.
Sea-Level Rise: Melting polar ice caps and glaciers, along with thermal expansion of seawater, contribute to rising sea levels, threatening coastal communities and ecosystems.
Ecosystem Disruption: Changes in temperature and precipitation affect habitats, leading to shifts in species distributions, altered migration patterns, and increased extinction rates.
Ocean Acidification: Increased
levels lead to higher absorption of carbon dioxide by oceans, lowering pH and harming marine life, particularly organisms with calcium carbonate shells or skeletons.
Human Health and Economy: Impacts on agriculture, infrastructure, water resources, and human health, including heat-related illnesses and the spread of vector-borne diseases.
Mitigation Efforts:
Reducing GHG Emissions: Transitioning to renewable energy sources, enhancing energy efficiency, and implementing carbon capture technologies.
Carbon Sequestration: Enhancing natural sinks like forests, wetlands, and oceans to absorb
CO2 from the atmosphere.
International Agreements: Global cooperation through treaties like the Paris Agreement aims to limit global temperature rise by setting emission reduction targets.
In summary, the greenhouse effect is essential for maintaining Earth’s habitable climate, but human-induced enhancements are disrupting this balance, leading to widespread environmental, economic, and health challenges. Addressing these issues requires concerted global efforts to reduce greenhouse gas emissions and adapt to the changing climate.
12. What is the role of DNA replication in heredity, and what mechanisms ensure its fidelity?
Answer: DNA Replication is the biological process by which a cell duplicates its DNA, ensuring that each daughter cell receives an identical copy of the genetic material. This process is fundamental to heredity, allowing genetic information to be transmitted from one generation to the next.
Role in Heredity:
Genetic Continuity: DNA replication ensures that genetic information is accurately passed from parent to offspring during cell division (mitosis in somatic cells and meiosis in gametes).
Variation Through Recombination: While replication aims for accuracy, mechanisms like crossing over during meiosis introduce genetic variation, which is essential for evolution and adaptation.
Cellular Function: Accurate replication is crucial for maintaining the integrity of the genome, enabling proper gene expression and cellular function.
Mechanisms Ensuring Fidelity:
Base Pairing Rules:
- Complementary Base Pairing: Adenine (A) pairs with thymine (T), and cytosine (C) pairs with guanine (G). Strict adherence to these rules minimizes errors during replication.
Enzymatic Proofreading:
- DNA Polymerase Proofreading Activity: DNA polymerases possess 3’ to 5’ exonuclease activity, allowing them to remove incorrectly paired nucleotides immediately after insertion.
- Error Correction: If a mismatched base is incorporated, the polymerase detects the distortion in the DNA helix, excises the wrong base, and replaces it with the correct one.
Mismatch Repair Systems:
- Post-Replication Correction: Additional repair mechanisms identify and correct mismatches that escape the proofreading activity, ensuring high accuracy in the final DNA sequence.
High-Fidelity DNA Polymerases:
- Selective Enzymes: Specialized DNA polymerases with low error rates are responsible for the bulk of DNA synthesis, reducing the likelihood of mutations.
Redundancy and Multiple Checks:
- Multiple Layers of Defense: The combination of proofreading, mismatch repair, and the inherent accuracy of base pairing provides redundancy, ensuring errors are caught and corrected efficiently.
Structural Features of DNA:
- Double Helix Stability: The helical structure allows for the detection of mismatches through physical distortions, facilitating repair mechanisms.
Consequences of Replication Errors:
Mutations: Uncorrected errors can lead to mutations, which may have neutral, beneficial, or detrimental effects on an organism.
Genomic Instability: Accumulation of mutations can result in genomic instability, potentially leading to diseases like cancer.
Significance of High Fidelity:
Genetic Integrity: Ensures that the genetic information remains stable across generations, preserving the functional traits of organisms.
Adaptation and Evolution: While high fidelity maintains genetic consistency, the occasional mutation introduces diversity necessary for evolution and adaptation to changing environments.
In summary, DNA replication is central to heredity, ensuring the faithful transmission of genetic information. Multiple mechanisms, including enzymatic proofreading, mismatch repair, and the inherent accuracy of base pairing, work in concert to maintain high fidelity during replication, safeguarding the integrity of the genome.
These thought-provoking questions and detailed answers cover a wide range of fundamental science topics, including biology, chemistry, physics, and environmental science. They are designed to deepen understanding, encourage critical thinking, and reinforce key concepts essential for academic success in STEM disciplines