Industrial Ecology and Circular Economy
Industrial Ecology and the Circular Economy represent a transformative shift in how we design, operate, and evaluate modern industrial systems. Rather than following the traditional linear model of “take, make, dispose,” these approaches advocate for regenerative systems in which resource use, waste generation, and environmental impact are continuously minimized. This systems-based thinking integrates ecological principles into industrial operations, encouraging closed-loop cycles where materials and energy are reused efficiently across various sectors. This holistic vision aligns strongly with the goals of Environmental Engineering, helping aspiring engineers understand the symbiotic relationship between technology and nature.
At the core of the Circular Economy is the concept of designing out waste. This begins with rethinking how products are conceptualized and manufactured, ensuring that every component is either recyclable, biodegradable, or reusable. One practical application lies in shifting to biodegradable polymers and bio-based materials that degrade harmlessly, reducing dependence on persistent plastics. These materials often find synergy with innovations in Air Quality Engineering, where low-emission materials and advanced filtration systems contribute to cleaner industrial zones and healthier urban air.
Beyond materials, circular thinking influences how systems are structured. For example, industrial symbiosis allows the waste or by-products of one process to become the feedstock for another. This is increasingly common in eco-industrial parks, where shared infrastructures for waste heat, materials exchange, or water reuse drive down emissions. These collective practices support national and international efforts to mitigate and adapt to climate change, as industries must play a leading role in reducing greenhouse gas emissions.
To support such complex systems, professionals must rely on accurate, real-time data to monitor flows and feedback. That’s where Environmental Monitoring and Data Analysis becomes indispensable. Tools such as lifecycle assessment (LCA), material flow analysis (MFA), and Geographic Information Systems (GIS) help map the impact of industrial operations over time and space. These data-driven approaches also inform regulations under Environmental Policy and Management, shaping laws that mandate emissions caps, recycling quotas, and reporting requirements.
One highly visible application of circular design can be seen in Green Building practices, where architects and engineers integrate sustainability into every aspect of construction—from site planning and materials selection to energy systems and end-of-life disassembly. This reduces both embodied carbon and operational emissions. Projects like these illustrate the systemic nature of circular thinking, combining disciplines to deliver performance and environmental benefit simultaneously. In fact, many cities now include circular design principles in building codes and urban planning guidelines, illustrating how widespread these practices are becoming across the built environment.
In parallel, clean energy systems support industrial circularity by decarbonizing production and logistics. Renewable Energy Systems Engineering facilitates the integration of solar arrays, wind farms, and bioenergy plants into factories, warehouses, and data centers. These distributed power sources help reduce dependency on fossil fuels and support the growing demand for low-carbon products. Many companies now pursue circularity targets aligned with global sustainability frameworks, underscoring the urgency and value of transitioning to closed-loop industrial systems.
Waste management is another foundational pillar of the circular economy, moving beyond simple disposal toward recovery, reuse, and reintegration. Through Waste Management Engineering, professionals develop methods for material separation, composting, anaerobic digestion, and thermochemical conversion. Advanced waste-to-energy plants, for instance, convert organic waste into electricity and heat while minimizing landfilling. These strategies not only lower the environmental burden but also create new economic value from discarded materials, reshaping how we define and manage waste across sectors.
Achieving these efficiencies requires rethinking manufacturing from the ground up. Knowledge in Industrial and Manufacturing Technologies enables engineers to redesign production systems that use fewer resources and generate less waste. For example, Additive Manufacturing (3D printing) allows for near-zero material waste, on-demand customization, and decentralized production, all of which align well with circular goals. Similarly, Advanced Materials like graphene composites or self-healing polymers extend product lifespans and reduce replacement needs.
Increasingly, digital technologies are enabling precise tracking and management of material life cycles. Digital Twin Technology allows for real-time simulations of entire production lines or supply chains, predicting when components will fail or require maintenance. This prevents premature replacement and supports proactive resource planning. Meanwhile, Computer-Integrated Manufacturing ensures seamless coordination between design, production, and quality control, reducing idle time and raw material use. The combination of these tools strengthens energy and resource efficiency throughout the manufacturing value chain.
In practice, applying circular principles at scale often involves streamlining processes for leaner outcomes. Lean Manufacturing contributes methodologies for eliminating unnecessary steps and materials, fostering a culture of continuous improvement. This minimizes overproduction, overprocessing, and underutilization of assets. In addition, integrating human factors ensures that circular systems remain practical and safe for operators, technicians, and maintenance personnel. It also promotes inclusion and skill development among the industrial workforce, enhancing long-term system resilience.
To maintain high standards while using recovered or remanufactured materials, stringent oversight is critical. Manufacturing Quality Control and Assurance guarantees that all products, regardless of origin, perform reliably and meet regulatory specifications. Advanced statistical tools, real-time defect tracking, and automated inspection systems are increasingly being used to ensure uniformity. These innovations build trust in recycled goods, making it easier for consumers and businesses to embrace circular solutions without compromising quality or performance.
The integration of smart systems under the umbrella of Smart Manufacturing and Industry 4.0 is rapidly transforming industrial ecology. Through AI, IoT sensors, and machine learning algorithms, industries can analyze real-time data to adjust operations dynamically—reducing energy use, predicting maintenance needs, and optimizing supply chains. This enables responsive manufacturing that adapts to environmental constraints and resource availability. The rise of self-regulating systems also introduces the potential for decentralized production nodes that support local economies while reducing transportation emissions and material redundancies.
The circular economy’s relevance extends beyond material recovery—it redefines the role of energy within industrial systems. The adoption of Renewable Energy Systems Engineering ensures that production cycles are powered by low-carbon, sustainable energy sources such as wind, solar, and bioenergy. Energy storage solutions like batteries and hydrogen cells further enable industries to decouple from fossil fuel dependency. These shifts are key to achieving decarbonized operations and aligning with international climate commitments such as the Paris Agreement, which has catalyzed global investment in energy efficiency and green infrastructure. For example, this overview of the circular economy by the Ellen MacArthur Foundation provides a comprehensive view of how industries are reengineering energy use to support regenerative models.
Water is another vital resource often overlooked in circular strategies. Through the principles of Water Resources Engineering, industries implement closed-loop water systems that recycle greywater, harvest rainwater, and reduce reliance on freshwater withdrawals. This is especially critical in arid regions or water-stressed industries like textiles, mining, and agriculture. Techniques such as membrane filtration, constructed wetlands, and zero-liquid discharge (ZLD) systems are increasingly being adopted to minimize water footprints while preserving surrounding ecosystems.
Industrial ecology also benefits from synergies with environmental systems thinking. This interdisciplinary framework views industries as nested within larger ecological and social systems, recognizing their mutual dependencies and shared limits. It promotes lifecycle assessments (LCA), ecological footprint analysis, and ecosystem service valuation as tools for evaluating the broader consequences of industrial activities. These metrics guide decision-makers in choosing materials, suppliers, and distribution strategies that align with both profitability and planetary health.
In summary, Industrial Ecology and the Circular Economy offer a transformative vision for rethinking how we design, produce, consume, and repurpose resources. By aligning engineering innovation with ecological intelligence, these disciplines promote industrial networks that are self-sustaining, adaptive, and resilient. From smart manufacturing to ecological restoration, from renewable energy integration to closed-loop water management, the transition to circular systems is no longer theoretical—it is already underway across the globe. For students, engineers, and policymakers alike, mastering these concepts is essential to building a future that thrives within the boundaries of nature.
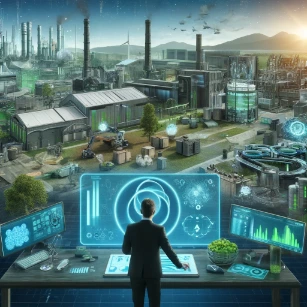
Table of Contents
Core Concepts in Industrial Ecology
Systems Thinking
Systems thinking is a foundational concept in industrial ecology, emphasizing the interconnection between industrial processes, environmental impacts, and socio-economic outcomes. Rather than examining a single component of a production system in isolation, systems thinking evaluates how all parts interact within a broader context. For example, a manufacturing facility’s energy use cannot be understood independently of its supply chain logistics, employee behavior, or waste management practices. This holistic perspective helps identify hidden inefficiencies and leverage points where small interventions can lead to significant improvements.
Systems thinking also allows engineers and sustainability professionals to assess unintended consequences and feedback loops. A change in raw material sourcing might reduce carbon emissions but increase water usage downstream—an insight only visible through system-level modeling. Tools like causal loop diagrams and stock-and-flow models enable visualization of these complex dynamics. Thwink.org offers valuable resources and examples for understanding how systems thinking applies to sustainability and industrial design.
Material and Energy Flow Analysis
Material Flow Analysis (MFA) and Energy Flow Analysis (EFA) are critical methodologies in industrial ecology for tracking the inputs, transformations, and outputs of resources. These analyses help visualize how raw materials, semi-finished goods, and waste products move through industrial systems. MFA, for instance, can reveal how aluminum extracted through bauxite mining is refined, used in products like cars or beverage cans, and ultimately collected for recycling. Understanding these flows allows industries to reduce waste and improve material efficiency by closing loops and designing products for recyclability.
Energy Flow Analysis complements MFA by detailing how energy is generated, consumed, and dissipated. This could include examining fossil fuel use in transportation, electricity consumption in manufacturing, or the recovery of waste heat through cogeneration. These insights support decarbonization strategies and inform energy policy. Advanced flow analysis often uses Sankey diagrams to visualize quantitative relationships between flows. When used together, MFA and EFA empower industries to make data-driven decisions that optimize sustainability and cost-effectiveness.
Life Cycle Assessment (LCA)
Life Cycle Assessment (LCA) is a systematic method for evaluating the environmental impacts associated with all stages of a product’s life—from raw material extraction, manufacturing, and usage to end-of-life disposal or recycling. This cradle-to-grave analysis is essential for identifying environmental “hotspots” and enabling comparisons between alternative production methods. For example, LCA might show that a paper bag, though biodegradable, may have a higher water footprint than a plastic bag when considering the entire life cycle.
LCA results often guide design decisions, corporate sustainability strategies, and public policy. Tools like GaBi and SimaPro provide standardized databases and modeling environments for LCA practitioners. ISO 14040/44 standards outline the general principles and framework for conducting robust LCAs. By using LCA, industries can align their operations with science-based sustainability targets, reduce hidden environmental costs, and build more transparent supply chains.
Industrial Symbiosis
Industrial symbiosis is a practice where different industries collaborate to utilize each other’s byproducts, energy, and resources, thus turning waste from one process into valuable input for another. This collaboration reduces environmental impact, minimizes raw material usage, and often results in economic savings for participating entities. The Kalundborg Symbiosis in Denmark remains a pioneering example, where power plants, pharmaceutical factories, and oil refineries exchange steam, fly ash, wastewater, and other byproducts to mutual benefit.
Key to the success of industrial symbiosis is proximity, trust, and the creation of a shared logistics and information system. Governments and regional development agencies can facilitate such exchanges by creating industrial parks designed around symbiotic principles. As digital platforms evolve, even small and geographically dispersed companies can identify potential partners for resource exchange, making symbiosis more scalable and efficient.
Core Concepts in Circular Economy
Principles of Circular Economy
The circular economy is a transformative model that challenges the traditional linear economy, which follows a ‘take-make-dispose’ trajectory. Instead, it offers a regenerative system where products, components, and materials maintain their highest utility and value at all times. It is built on three foundational principles that work together to form a cohesive sustainability strategy: designing out waste and pollution, keeping products and materials in use, and regenerating natural systems.
- Design Out Waste and Pollution:
- At the heart of this principle is the proactive effort to prevent waste and pollution by addressing them at the design stage. Engineers, product designers, and manufacturers integrate sustainability from the beginning by selecting non-toxic materials, reducing material diversity, and simplifying disassembly. For instance, using mono-material packaging ensures recyclability and minimizes contamination. By embedding environmental concerns in the design process, this principle reduces the systemic risks of pollution and mitigates ecological degradation.
- Industries also leverage digital technologies such as AI-driven simulation tools to evaluate environmental impact scenarios before committing to a design, ensuring that waste reduction is optimized throughout the product lifecycle.
- Keep Products and Materials in Use:
- This principle emphasizes the maximization of material and product utility through practices such as reuse, refurbishment, remanufacturing, and recycling. Products are designed for longevity, with modular components that are easy to repair or upgrade. A shift toward leasing or product-as-a-service models, especially in electronics and mobility sectors, further supports this principle by incentivizing producers to maintain products efficiently over extended periods.
- In developed circular systems, entire product categories are supported by take-back programs. For example, some clothing brands now allow customers to return worn garments, which are then refurbished, resold, or recycled into new fabric. This circular loop enhances resource efficiency and supports new business opportunities that align with sustainability goals.
- Regenerate Natural Systems:
- Rather than merely minimizing harm, this principle aims to create net positive environmental outcomes. Agricultural and industrial processes are redesigned to support soil health, biodiversity, and ecosystem services. Regenerative agricultural practices such as cover cropping, agroforestry, and composting return nutrients to the soil while sequestering carbon.
- Industries implementing circular strategies use renewable energy sources and shift toward biodegradable inputs. For example, bioplastics made from cornstarch or algae serve as compostable alternatives to petroleum-based plastics, ensuring that materials flow back into the biosphere harmlessly. Further insights into regenerative systems and circular design integration are discussed in the Ellen MacArthur Foundation’s overview on circular economy.
Circular Design Strategies
Circular design strategies form the operational backbone of the circular economy. These strategies ensure that products, buildings, and systems are created with an inherent ability to fit into closed-loop cycles. Such designs consider the entire lifecycle from material extraction to end-of-life disposal, emphasizing ease of disassembly, reuse, and recycling.
- Cradle-to-Cradle Design:
- Cradle-to-cradle is a revolutionary approach to product design where every component is conceived as either biodegradable or infinitely recyclable. Inspired by nature, it eliminates the concept of waste entirely. For example, carpet tiles designed for cradle-to-cradle recycling use safe dyes and materials that can be recovered and remanufactured into new tiles without loss in quality or toxicity concerns.
- Manufacturers embracing this strategy also conduct rigorous certification processes to ensure that their products meet high standards of environmental safety, material health, and social fairness.
- Modular Design:
- Modular design empowers users to repair or upgrade specific parts of a product without replacing the entire item. This is especially relevant in electronics and furniture design. Smartphones with swappable batteries or camera modules exemplify this strategy, reducing electronic waste and extending the device’s useful life.
- Additionally, in architecture, modular buildings allow entire units or panels to be reused in new constructions, facilitating adaptive reuse and minimizing demolition waste.
- Biomimicry:
- Biomimicry involves designing processes, products, and systems inspired by natural cycles and organisms. By mimicking efficient natural strategies—such as spider silk for material strength or termite mounds for passive cooling—engineers develop innovations that are energy-efficient, resilient, and sustainable.
- These designs often result in multi-functional systems that adapt to changing conditions and foster resource regeneration, positioning them as ideal candidates for circular economy implementation.
Resource Loops
Resource loops ensure that materials continue circulating within the economy rather than being discarded as waste. The design and implementation of these loops depend on separating biological and technical cycles so that each type of material is processed correctly. Closed-loop systems reduce the extraction of virgin resources and lower the environmental footprint of production and consumption activities.
- Technical Loop:
- Technical materials—such as metals, glass, and most plastics—can be recovered and reprocessed into new products without a loss of quality. Industrial symbiosis plays a key role in establishing these loops, where waste or byproducts from one manufacturer become the raw materials for another. For example, aluminum cans can be recycled repeatedly, consuming only 5% of the energy required to produce them from raw bauxite.
- Companies often employ extended producer responsibility (EPR) schemes to reclaim and reintegrate their materials, enhancing accountability and fostering circular business practices.
- Biological Loop:
- Organic materials such as food scraps, wood, and paper are processed through composting, anaerobic digestion, or enzymatic breakdown. The result is soil enrichment, energy generation, and closed nutrient cycles. For example, food waste from urban households can be composted and returned to peri-urban farms as fertilizer, establishing a continuous cycle that supports both agriculture and waste reduction.
- This loop is vital for maintaining healthy ecosystems, as it ensures that biological nutrients are returned to the environment in a form that supports future growth.
Applications of Industrial Ecology and Circular Economy
Sustainable Manufacturing
- Material Efficiency:
- Using fewer raw materials and maximizing resource utilization through improved product design, process optimization, and substitution with sustainable alternatives.
- Techniques such as lightweighting, modular design, and precision manufacturing help reduce raw material inputs while maintaining product integrity and functionality.
- Digital twins and simulation tools enable predictive adjustments to minimize excess consumption.
- Lean manufacturing practices contribute to higher yield rates and lower scrap generation.
- Waste Reduction:
- Implementing closed-loop production systems where waste is reused as input for other operations within or across industrial facilities.
- Zero-waste factories aim to completely eliminate landfill contributions by repurposing byproducts, introducing take-back schemes, and enhancing end-of-life processing.
- Improved segregation, tracking, and reuse of industrial residues enhances environmental performance and reduces disposal costs.
- Collaborative networks allow one company’s output waste to become another’s valuable input.
- Renewable Energy Integration:
- Powering industrial operations with wind, solar, or hydropower reduces dependency on fossil fuels and associated greenhouse gas emissions.
- Microgrids, smart inverters, and energy storage systems increase resilience and allow seamless integration of renewables into production cycles.
- Real-time energy analytics help match demand to green energy supply and optimize equipment scheduling.
- Onsite renewable installations reduce transmission losses and bolster sustainability branding.
Urban and Regional Planning
- Eco-Industrial Parks:
- Clusters of businesses that share resources and byproducts to create synergies, increase efficiency, and reduce collective environmental impact.
- Examples include heat exchange between power plants and greenhouses, water reuse across manufacturing facilities, and shared logistics infrastructure.
- Such parks encourage co-location, which reduces transportation emissions and promotes systemic collaboration.
- Policies supporting zoning flexibility and tax incentives further accelerate development of these industrial ecosystems.
- Circular Cities:
- Urban areas designed with circular principles, focusing on resource reuse, green infrastructure, and local food systems.
- Key strategies include compact urban design, water-sensitive development, community composting, and renewable energy microgrids.
- Circular cities leverage citizen engagement, decentralized technologies, and local governance to implement sustainability objectives.
- Initiatives like the Circular Glasgow project showcase scalable models for systemic transformation of urban areas as highlighted by the Ellen MacArthur Foundation.
Resource Recovery and Recycling
- E-Waste Recycling:
- Recovering precious metals and rare earth elements from discarded electronics through processes like mechanical separation, hydrometallurgy, and pyrometallurgy.
- Certified e-waste facilities ensure proper handling of hazardous components and enhance recovery efficiency.
- Consumer incentive programs encourage the return of old devices for responsible recycling.
- Urban mining contributes to resource security by tapping into existing waste streams rather than virgin extraction.
- Industrial Byproducts:
- Using slag from steel production in road construction or as a cement additive, thus diverting millions of tons of waste from landfills annually.
- Fly ash, gypsum, and biomass ash are being explored for their pozzolanic and structural properties in green construction materials.
- Standardized classification and quality control protocols are critical for market uptake.
- Byproduct valorization supports both cost savings and environmental compliance.
- Textile Recycling:
- Transforming used fabrics into new materials for the fashion industry through fiber regeneration, chemical recycling, and mechanical shredding.
- Blended and synthetic fabrics pose technical challenges, but innovations in enzymatic separation and polymer recovery are improving recyclability.
- Retailers increasingly offer garment collection bins and secondhand resale platforms as part of circular apparel strategies.
- Designing for disassembly and mono-material garments can significantly boost textile circularity.
Waste-to-Energy Systems
- Anaerobic Digestion:
- Converting organic waste into biogas and nutrient-rich digestate using microbial processes in oxygen-free environments.
- Municipal food waste, agricultural residues, and sludge from wastewater treatment plants are common feedstocks.
- Biogas can be used for electricity, heat, or upgraded to biomethane for injection into natural gas grids.
- Digestate is processed into biofertilizers, returning nutrients to soil systems.
- Thermal Conversion:
- Using pyrolysis or gasification to produce energy from non-recyclable waste materials such as contaminated plastics or composite materials.
- These high-temperature, low-oxygen methods convert solids into syngas, bio-oil, and char.
- Advanced emission controls and energy recovery technologies make modern thermal conversion a low-impact alternative to landfilling.
- Policy frameworks and carbon credit schemes can incentivize adoption of waste-to-energy plants.
Circular Supply Chains
- Reverse Logistics:
- Systems for collecting and returning used products for repair, refurbishment, or recycling, enabling manufacturers to reclaim valuable components and reduce environmental impact.
- Barcode and RFID tracking facilitate efficient return flows and quality assurance.
- Returned goods may be reconditioned for resale, stripped for parts, or responsibly dismantled for material recovery.
- Strong customer engagement and transparent return policies are key enablers of reverse logistics success.
- Sharing Platforms:
- Encouraging shared ownership of goods, such as cars or equipment, to reduce demand for new production and maximize utilization rates.
- Digital platforms coordinate availability, access, payment, and maintenance of shared assets.
- Examples include car-sharing apps, tool libraries, co-working spaces, and peer-to-peer rental systems.
- Shared economy models extend product life and displace resource-intensive alternatives.
Food Systems
- Reducing Food Waste:
- Optimizing supply chains to prevent spoilage and redistributing surplus food to food banks and shelters.
- Smart packaging, cold chain monitoring, and dynamic pricing reduce waste across retail and hospitality sectors.
- Public awareness campaigns, food waste audits, and date labeling reforms further decrease edible waste volumes.
- Edible byproducts can be repurposed into animal feed or functional food ingredients.
- Composting:
- Converting food waste into organic fertilizer through aerobic decomposition processes in centralized or decentralized composting systems.
- Compost enriches soil, enhances water retention, and sequesters carbon, contributing to regenerative agriculture.
- Proper segregation and contamination control are essential for high-quality compost production.
- Urban rooftop farms and community gardens benefit directly from locally produced compost.
- Urban Agriculture:
- Growing food locally using recycled water and organic waste as inputs, enhancing food security and reducing transportation emissions.
- Techniques include hydroponics, aquaponics, and vertical farming, often integrated into buildings and vacant spaces.
- Urban farms supply fresh produce to local markets and promote education on sustainable food systems.
- Policy support and community participation are vital for scaling urban agriculture initiatives.
Emerging Technologies in Industrial Ecology and Circular Economy
Digital Tools and Big Data
- IoT Sensors:
- Real-time monitoring of resource flows in industrial systems has become more advanced through the deployment of IoT sensors. These devices are embedded in equipment and pipelines to capture data on energy consumption, water usage, emissions, and material throughput. For example, in a manufacturing plant, IoT-enabled meters can track the exact quantity of steam used in various operations and detect leaks or inefficiencies in real time.
- In circular economy models, IoT facilitates traceability of materials through their lifecycle, enabling manufacturers to optimize logistics and plan for disassembly, reuse, or recycling. Sensors embedded in products can also help consumers monitor durability and trigger maintenance or return alerts before failure occurs, extending product life cycles.
- AI and Machine Learning:
- Artificial intelligence (AI) and machine learning (ML) algorithms are revolutionizing waste sorting and recycling efficiency. Smart waste-sorting machines use computer vision and deep learning to differentiate between types of plastics or metals at high speed, improving the purity of recycled materials.
- In addition, AI is deployed for predictive maintenance, energy optimization, and anomaly detection within circular production systems. Predictive algorithms help forecast equipment wear or performance drops, allowing for proactive adjustments. These systems also contribute to smart inventory and material flow management, reducing overproduction and waste.
- According to World Economic Forum, AI technologies are poised to unlock up to $127 billion annually in material savings and economic value in circular economy transitions.
- Blockchain Technology:
- Blockchain ensures transparency and traceability in circular supply chains by recording every transaction and transformation a material undergoes. This is particularly useful in tracking recycled content, ethical sourcing, and product certifications.
- Decentralized blockchain networks are being used to verify claims of sustainability and ensure compliance with environmental regulations. They also provide secure frameworks for product-as-a-service models, enabling efficient resource sharing and end-of-life product tracking.
- For example, blockchain-enabled digital product passports can store maintenance records, ownership history, and component breakdowns, aiding efficient reuse and recycling.
Advanced Recycling Technologies
- Chemical Recycling:
- Chemical recycling breaks down polymers into their monomers, allowing for high-quality, virgin-like plastic recovery. Unlike mechanical recycling, which degrades material quality, chemical methods can handle contaminated or mixed plastic waste streams.
- Technologies such as pyrolysis and depolymerization allow for closed-loop systems where plastic packaging or textiles can be continuously recycled without quality loss. These processes are increasingly integrated into large-scale waste treatment plants.
- Hydrometallurgy:
- Hydrometallurgical techniques are gaining traction in recovering critical raw materials from e-waste, such as lithium, cobalt, and rare earth elements. The method uses aqueous solutions to extract metals without the high energy demands of smelting.
- This approach not only reduces environmental impact but also supports domestic supply chains for green technologies, especially for electric vehicle batteries and renewable energy components. Research institutions and startups are innovating to reduce reagent usage and improve metal recovery efficiency.
Biobased Materials
- Bioplastics:
- Bioplastics are derived from renewable sources such as corn starch, sugarcane, or algae and are designed to replace petroleum-based plastics. These materials can be biodegradable, compostable, or recyclable, depending on formulation.
- Industries are exploring the application of bioplastics in packaging, agriculture, automotive interiors, and even electronics. Advances in microbial engineering have led to new polymers like PHA and PLA with better performance and biodegradability profiles.
- Biosynthetic Alternatives:
- Biosynthetics involve the production of materials such as silk, leather, and rubber through microbial fermentation or plant-based processes. These alternatives reduce reliance on animal or petrochemical sources and can be engineered for circular end-of-life scenarios.
- Companies are developing biosynthetic foams, coatings, and adhesives with reduced carbon footprints and enhanced recyclability. These innovations align with regenerative design principles and are beginning to scale in commercial applications.
Additive Manufacturing (3D Printing)
- On-Demand Production:
- 3D printing enables localized, just-in-time manufacturing, reducing transportation needs and material wastage. It allows for the production of complex parts using minimal raw material and without the need for molds or excess tooling.
- Companies use digital design libraries and cloud-based manufacturing platforms to customize parts and print only what is needed, reducing overproduction and obsolescence. This on-demand model supports sustainable product development and inventory control.
- Recyclable Filaments:
- Recyclable or biodegradable filaments made from PLA, PETG, or recycled plastics enable closed-loop printing processes. Desktop recycling units can grind old prints and remold them into new filament spools, fostering maker-driven circularity.
- Research is advancing toward multi-material printing with embedded recycling markers, allowing printed products to be easily sorted and reintegrated into circular supply chains.
Renewable Energy Integration
- Circular Energy Systems:
- Industrial ecology benefits from the integration of renewable energy sources like wind, solar, and bioenergy. These inputs power processes that are designed to minimize emissions and utilize waste heat or byproducts as secondary resources.
- Energy management platforms analyze demand profiles and adjust supply schedules to optimize energy use. Some circular factories employ waste-to-energy systems to convert organic residues into electricity or heat, creating self-sustaining energy loops.
- Partnerships between renewable energy providers and industrial parks are fostering shared infrastructure, distributed generation, and long-term energy contracts that stabilize costs and reduce carbon footprints.
Challenges in Implementing Industrial Ecology and Circular Economy
- Economic Feasibility:
- The initial capital investment for transitioning to circular models, including advanced recycling infrastructure, renewable energy integration, and sustainable design practices, can be prohibitively high for many firms, particularly small and medium-sized enterprises (SMEs).
- Access to funding and financing mechanisms is often limited, especially in developing regions, where governments may prioritize immediate economic growth over long-term sustainability.
- The payback period for circular economy investments tends to be longer, which can deter traditional investors seeking quick returns.
- Moreover, the lack of standardized metrics to quantify economic benefits—such as avoided resource depletion or reduced emissions—complicates efforts to demonstrate financial viability to stakeholders.
- Innovative public-private partnerships and green financing models are being explored to bridge this gap, but widespread adoption remains a challenge.
- Regulatory Barriers:
- In many jurisdictions, regulations have not yet caught up with the principles of the circular economy. Existing environmental and industrial policies often support linear practices—such as landfilling and incineration—over reuse and recycling.
- There is a lack of cohesive international frameworks that promote material reuse across borders, creating trade obstacles for refurbished goods and secondary raw materials.
- Complex and sometimes conflicting legislation—particularly around waste classification, chemical safety, and end-of-life product responsibility—can discourage circular innovation.
- Streamlined regulations and supportive legislation such as extended producer responsibility (EPR) laws are needed to incentivize circular product design and recycling initiatives.
- Consumer Behavior:
- Despite increasing awareness of environmental issues, consumer habits often prioritize convenience, low cost, and novelty over sustainability.
- Many consumers are unfamiliar with circular economy concepts like product-as-a-service models, leasing, or modular product repairability.
- Perceived lower quality or stigma associated with recycled or refurbished products can inhibit their market acceptance.
- Behavioral change requires sustained educational campaigns, transparent product labeling, and incentives such as deposit-return schemes or discounts on refurbished goods.
- Studies such as those highlighted by OECD’s research on environmental behavioral insights reveal that social norms and default options significantly influence sustainable choices.
- Technological Gaps:
- While technologies like chemical recycling, biorefineries, and digital traceability tools are emerging, many are still in the early stages of commercialization or lack the scalability needed for broad implementation.
- There is insufficient infrastructure to process complex waste streams, such as composite materials or mixed e-waste, in an economically viable and environmentally sound manner.
- Disruptive innovation is required not only in materials and processes, but also in digital platforms that support circular marketplaces and reverse logistics coordination.
- Tech transfer mechanisms between research institutions and industry remain underdeveloped in many countries, leading to a gap between innovation and practical deployment.
- Supply Chain Complexity:
- Modern global supply chains are deeply entrenched in linear modes of production and consumption, making the transition to circular systems highly complex.
- It can be difficult to track material flows and ensure product traceability across multiple tiers of suppliers, especially when dealing with imported goods or outsourced production.
- Reverse logistics—essential for collecting, sorting, and reprocessing used products—requires coordination among manufacturers, retailers, consumers, and recycling operators.
- Digital tools like blockchain and IoT devices can help overcome traceability barriers, but their adoption remains uneven across sectors and regions.
- Building resilient, transparent, and circular supply chains demands not only technological investment but also trust, cooperation, and aligned incentives among all stakeholders.
Case Studies in Industrial Ecology and Circular Economy
Kalundborg Eco-Industrial Park, Denmark
- Kalundborg is often cited as the world’s first functioning example of an industrial symbiosis network, where multiple companies collaborate to exchange waste materials and energy in a closed-loop manner.
- The park includes power plants, pharmaceutical companies, oil refineries, and municipal services, all of which are interconnected by a complex web of material and energy flows.
- Excess heat from a power station is piped to nearby homes and public buildings for district heating, while steam is shared between industrial partners to reduce energy consumption and costs.
- Other exchanges include fly ash from combustion processes used in cement production, sludge from biotech processes turned into fertilizer, and cooling water shared between facilities.
- This symbiotic model has led to significant reductions in greenhouse gas emissions, water usage, and raw material consumption, while fostering community development and economic resilience.
- The success of Kalundborg has inspired similar projects globally and demonstrated how voluntary cooperation among industries can lead to sustainable innovation without requiring top-down mandates.
Ellen MacArthur Foundation Initiatives
- The Ellen MacArthur Foundation is a leading global advocate for the circular economy, engaging businesses, policymakers, and educators to shift toward regenerative and restorative systems.
- One of its major initiatives, the New Plastics Economy, brings together more than 1,000 organizations to rethink plastic packaging design, production, and after-use pathways to eliminate pollution.
- The foundation partners with global brands such as Unilever, IKEA, and Google to integrate circular design principles into their products and operations.
- Its Circular Economy 100 (CE100) network facilitates cross-sector collaboration, helping organizations pilot circular solutions and scale their impact through shared learning and innovation labs.
- It has also collaborated with major academic institutions to produce cutting-edge research and educational resources that influence curriculum design and policymaking worldwide.
- One key resource, the Ellen MacArthur Foundation Resource Library, provides case studies, tools, and reports to support global circular transformation.
Closed-Loop Recycling in the Automotive Industry
- Automotive manufacturers have increasingly embraced closed-loop recycling strategies to reduce their environmental footprint and material costs.
- BMW has implemented a circular material cycle for aluminum, where offcuts from vehicle production are collected and reintroduced into the manufacturing process, conserving energy and resources.
- Similarly, Tesla’s Gigafactories aim to recycle used battery packs to recover valuable metals like lithium, nickel, and cobalt, closing the loop on electric vehicle (EV) production.
- Automakers also collaborate with suppliers to design vehicle components—such as bumpers and dashboards—from recycled plastics, making use of post-consumer and post-industrial waste.
- Recyclability is now a consideration from the early stages of vehicle design, with modular architectures that enable disassembly and materials recovery at end-of-life.
- Regulatory incentives and extended producer responsibility programs in regions like the EU further support these closed-loop practices by requiring manufacturers to meet recycling and recovery targets.
Future Directions in Industrial Ecology and Circular Economy
- Global Adoption of Circular Policies:
- As climate change and resource depletion intensify, global institutions and governments are increasingly aligning with circular economy principles. Policy frameworks are evolving to support waste minimization, extended producer responsibility, and eco-design standards.
- At the international level, organizations such as the United Nations and OECD have issued guidelines for integrating circularity into national strategies, influencing trade, taxation, and procurement policies.
- Examples include the European Union’s Circular Economy Action Plan, which mandates targets for recycling, reuse, and sustainable product design across multiple sectors.
- Countries like Japan and the Netherlands are global pioneers, embedding circular metrics into urban planning and industrial development, fostering transnational learning and cooperation.
- Widespread policy adoption is expected to incentivize investment in circular infrastructure and promote global markets for secondary materials, enhancing economic resilience and environmental stewardship.
- Circular Business Models:
- Future economic models will increasingly prioritize services over ownership, a shift that encourages resource efficiency and lifecycle responsibility. Leasing, product-as-a-service, and pay-per-use models reduce the incentive for overproduction and planned obsolescence.
- Companies are reimagining their value propositions by offering long-term maintenance, refurbishment, and upgrade services, extending product life while retaining customer loyalty.
- Digital technologies enable real-time monitoring of assets, facilitating predictive maintenance and improved usage efficiency. This opens up opportunities in sectors from electronics to fashion and construction.
- Subscription models for furniture, electronics, or even clothing are gaining traction, particularly among younger consumers concerned with sustainability.
- The Harvard Business Review highlights how circular business models are driving innovation and disrupting traditional linear supply chains.
- Urban Mining:
- As cities become more densely populated and technologically integrated, they accumulate vast quantities of valuable materials embedded in infrastructure, electronics, and consumer goods.
- Urban mining refers to the process of extracting raw materials—such as rare earths, copper, and gold—from discarded urban products rather than relying on virgin mining operations.
- High-rise buildings, outdated cables, e-waste, and demolished construction sites are all rich sources of materials that can be recovered and reused.
- This approach not only reduces dependence on traditional mining—which is often energy-intensive and environmentally destructive—but also lowers the carbon footprint associated with material extraction and processing.
- Advanced robotics, AI, and separation technologies are being developed to scale urban mining safely and cost-effectively, unlocking a new stream of circular resources.
- Education and Advocacy:
- The next generation of engineers, designers, and policymakers must be equipped with a circular mindset to lead sustainability transitions. Educational institutions are beginning to embed industrial ecology and circular economy concepts across curricula.
- Multidisciplinary programs are combining environmental science, systems thinking, and business strategy to foster innovative problem-solving around resource management.
- Public awareness campaigns, social media activism, and nonprofit initiatives play a critical role in shaping consumer habits and pressuring industries to adopt circular practices.
- Collaborations between academia, government, and industry are facilitating community workshops and knowledge-sharing platforms to accelerate adoption and innovation.
- Education is increasingly seen as a strategic enabler of systemic change toward a regenerative economy that respects planetary boundaries and social equity.
- Net-Zero Waste Systems:
- Achieving net-zero waste is an ambitious but attainable goal that requires redesigning entire industrial ecosystems to eliminate the concept of waste altogether.
- It involves closed-loop systems where all outputs—whether gases, solids, or liquids—are either recirculated as inputs or safely returned to the biosphere.
- Technological innovations such as carbon capture, enzymatic recycling, and smart manufacturing contribute to reducing emissions and conserving materials.
- Industrial parks and urban zones are being piloted with integrated utilities, real-time monitoring, and circular logistics to support zero-waste objectives.
- Net-zero waste is not only an environmental goal but also an economic one—reducing input costs, improving operational efficiency, and opening up green job markets in recycling and remanufacturing.
Why Study Industrial Ecology and Circular Economy
Redesigning Systems for Sustainability
Industrial ecology studies the flow of materials and energy through industrial systems. Students learn how to close loops and reduce waste by redesigning processes. This supports the shift from a linear to a circular economy.
Life-Cycle Thinking and Material Flows
Students analyze how products are made, used, and disposed of across their life cycle. They use tools like material flow analysis and life-cycle assessment. These insights inform more sustainable production and consumption systems.
Symbiosis Between Industries and Sectors
The course explores how waste from one industry can become a resource for another. Students study case studies of eco-industrial parks and circular supply chains. These strategies improve resource efficiency and competitiveness.
Policy, Innovation, and Business Models
Students examine how regulation, design, and entrepreneurship support the circular economy. They learn how to develop products, services, and systems that minimize resource extraction. This creates economic value while protecting the environment.
Systems Thinking for Global Sustainability
Industrial ecology emphasizes interconnectedness and long-term thinking. Students are trained to identify leverage points and systemic solutions. This prepares them to lead sustainability transitions in diverse contexts.
Industrial Ecology and Circular Economy: Conclusion
Industrial ecology and the circular economy represent transformative paradigms for navigating the complex interplay between industrial development, environmental stewardship, and long-term sustainability. These interconnected frameworks challenge the traditional “take-make-dispose” model that has dominated global production systems since the Industrial Revolution, offering instead a vision of cyclical resource use, regenerative systems, and collaborative innovation. Rooted in scientific, engineering, and socio-economic disciplines, they emphasize minimizing waste, extending product lifecycles, and designing ecosystems that mirror the efficiency and balance of natural systems.
By incorporating principles of systems thinking, industrial ecology enables organizations to map out the entire lifecycle of materials and energy, from extraction to end-of-life, revealing inefficiencies and intervention points. Circular economy strategies complement this analysis by providing actionable models such as product-as-a-service, remanufacturing, and regenerative agriculture, which can be adapted across various industries. The alignment of these approaches fosters the development of industrial symbiosis—whereby waste streams from one process serve as resources for another—creating economic savings while reducing environmental burdens.
Moreover, as sustainability transitions become a policy imperative, governments and corporations are turning to industrial ecology and circular economy tools to comply with regulatory targets, reduce carbon emissions, and enhance resilience to resource volatility. International accords such as the European Green Deal and UN Sustainable Development Goals (SDGs) have institutionalized the importance of circularity in global development agendas. Cities, too, are reimagining themselves as “circular hubs,” implementing initiatives like zero-waste districts, decentralized recycling infrastructures, and circular procurement systems.
Importantly, digital technologies such as artificial intelligence, Internet of Things (IoT), and blockchain are accelerating the adoption of circular strategies by enabling real-time monitoring, data sharing, and life-cycle transparency. These innovations are crucial in scaling circular operations from niche pilot projects to systemic change. According to the Ellen MacArthur Foundation, digitization plays a pivotal role in unlocking new value chains and optimizing circular logistics across global markets.
As industries confront the mounting pressures of climate change, biodiversity loss, and finite resource availability, industrial ecology and circular economy principles offer a coherent, integrative roadmap toward decarbonization and sustainable growth. Their adoption fosters not only environmental preservation but also economic competitiveness and social well-being by catalyzing green innovation, enhancing supply chain resilience, and empowering communities to rethink consumption patterns.
Ultimately, the successful implementation of these frameworks will depend on cross-sector collaboration, bold leadership, and a cultural shift in how value is defined and measured. Education, policy reform, stakeholder engagement, and investment in circular infrastructure must converge to institutionalize these practices across geographies and scales. In doing so, societies can transition from linear degradation to regenerative prosperity, ensuring a viable and thriving future for generations to come.
Industrial Ecology and Circular Economy: Review Questions with Detailed Answers:
1. What is industrial ecology and how does it contribute to environmental engineering?
Answer:
Industrial ecology is a multidisciplinary field that studies the flow of materials and energy through industrial systems with the goal of optimizing resource use and minimizing environmental impact. It draws inspiration from natural ecosystems to create sustainable industrial processes where waste from one process becomes input for another, thus promoting a closed-loop system.
Contribution to Environmental Engineering:
- Resource Optimization: Enhances the efficient use of materials and energy, reducing waste and conserving natural resources.
- Waste Minimization: Transforms waste into valuable resources, thereby decreasing the environmental burden of disposal.
- System Integration: Promotes the integration of various industrial processes to create symbiotic relationships, improving overall system sustainability.
- Life Cycle Thinking: Encourages considering the entire lifecycle of products and processes, leading to more sustainable design and operation.
- Innovation: Drives the development of new technologies and practices that support sustainable industrial practices.
Example:
A manufacturing plant implements an industrial ecology approach by using waste heat from one process to power another, thereby reducing overall energy consumption and minimizing thermal pollution.
2. Define the circular economy and explain its key principles.
Answer:
The circular economy is an economic system aimed at eliminating waste and the continual use of resources through principles of designing out waste, keeping products and materials in use, and regenerating natural systems. It contrasts with the traditional linear economy, which follows a ‘take-make-dispose’ model.
Key Principles:
- Design Out Waste and Pollution: Create products and processes that minimize waste generation and environmental impact.
- Keep Products and Materials in Use: Extend the lifecycle of products through reuse, repair, refurbishment, and recycling.
- Regenerate Natural Systems: Ensure that economic activities enhance rather than degrade natural ecosystems, promoting biodiversity and soil health.
Additional Principles:
- Systemic Thinking: Viewing the economy as interconnected systems to optimize resource flows.
- Value Creation: Focusing on maximizing the value derived from resources by keeping them circulating in the economy.
- Collaboration: Encouraging partnerships across industries to create symbiotic relationships that support circular practices.
Example:
A company designs its packaging to be easily recyclable and encourages customers to return used packaging for reuse or recycling, thereby reducing landfill waste and promoting material reuse.
3. How does the concept of “closing the loop” apply to the circular economy in industrial processes?
Answer:
“Closing the loop” in the circular economy refers to creating a closed-loop system where materials and products are continuously reused, recycled, or repurposed, eliminating the concept of waste. This approach ensures that resources are kept in circulation for as long as possible, reducing the need for virgin materials and minimizing environmental impact.
Application to Industrial Processes:
- Recycling: Processes waste materials into new products, reducing the need for raw material extraction.
- Remanufacturing: Restores used products to a like-new condition for resale, extending their lifecycle.
- Product-as-a-Service: Shifts the business model from selling products to providing services, incentivizing durability and maintenance.
- Biological Cycles: Uses biological materials that can safely return to the environment, promoting natural regeneration.
Benefits:
- Resource Efficiency: Maximizes the use of existing materials, reducing dependency on finite resources.
- Cost Savings: Lowers material costs through recycling and reuse, enhancing economic efficiency.
- Environmental Protection: Decreases pollution and greenhouse gas emissions by reducing waste and conserving resources.
- Innovation: Encourages the development of new technologies and business models that support circular practices.
Example:
An electronics manufacturer adopts a closed-loop system by taking back old devices, refurbishing them, and reselling them, thereby reducing electronic waste and conserving valuable materials like metals and plastics.
4. What are the main challenges in implementing circular economy practices in industrial settings?
Answer:
Implementing circular economy practices in industrial settings presents several challenges, including:
- Design Complexity: Designing products for longevity, repairability, and recyclability requires significant changes in product design and manufacturing processes.
- Economic Barriers: Initial investment costs for circular technologies and processes can be high, and the financial benefits may not be immediately apparent.
- Supply Chain Integration: Coordinating across multiple stakeholders and integrating circular practices into existing supply chains can be complex and resource-intensive.
- Technological Limitations: Lack of advanced recycling technologies and infrastructure can hinder the efficient recovery and reuse of materials.
- Regulatory and Policy Gaps: Inconsistent or inadequate regulations and policies may not support or incentivize circular practices effectively.
- Consumer Behavior: Shifting consumer mindset towards valuing sustainability and participating in circular practices like product take-back and recycling can be challenging.
- Information and Knowledge Gaps: Limited awareness and understanding of circular economy principles among businesses and employees can impede implementation.
Strategies to Overcome Challenges:
- Innovation in Design: Investing in research and development to create products that are easier to reuse, repair, and recycle.
- Economic Incentives: Providing subsidies, tax breaks, and other financial incentives to lower the cost barriers for adopting circular practices.
- Collaboration: Building partnerships across industries and sectors to share resources, knowledge, and infrastructure.
- Advancing Technology: Investing in and developing new technologies that facilitate material recovery and recycling.
- Policy Support: Advocating for supportive regulations and standards that encourage circular economy practices.
- Education and Awareness: Conducting training programs and awareness campaigns to educate stakeholders about the benefits and methods of implementing circular economy practices.
Example:
A manufacturing company faces high initial costs in redesigning its products for recyclability. To overcome this, it collaborates with suppliers and customers to develop a shared recycling infrastructure, reducing individual investment burdens and fostering a collaborative approach to circularity.
5. Explain the role of lifecycle assessment (LCA) in industrial ecology and the circular economy.
Answer:
Lifecycle Assessment (LCA) is a systematic methodology used to evaluate the environmental impacts of a product, process, or system throughout its entire lifecycle—from raw material extraction and manufacturing to use, maintenance, and disposal. In industrial ecology and the circular economy, LCA plays a critical role in identifying opportunities for reducing environmental impacts and enhancing sustainability.
Role of LCA:
- Impact Identification: Identifies the stages of a product’s lifecycle that have the most significant environmental impacts, allowing for targeted improvements.
- Decision Support: Provides data-driven insights to support decision-making in design, material selection, and process optimization.
- Performance Benchmarking: Enables comparison of different products or processes based on their environmental performance, fostering competition and innovation towards sustainability.
- Circular Strategy Development: Assists in designing circular strategies by evaluating the potential benefits and trade-offs of different reuse, recycling, and remanufacturing options.
- Transparency and Reporting: Facilitates transparent reporting of environmental performance, supporting certifications and regulatory compliance.
Benefits in Industrial Ecology and Circular Economy:
- Resource Efficiency: Helps in optimizing resource use by identifying and minimizing waste and inefficiencies throughout the lifecycle.
- Reduced Environmental Footprint: Guides the implementation of practices that lower greenhouse gas emissions, energy consumption, and pollution.
- Sustainable Design: Informs the design of products and systems that are easier to reuse, repair, and recycle, aligning with circular economy principles.
- Continuous Improvement: Encourages ongoing evaluation and improvement of processes and products to enhance sustainability over time.
Example:
An LCA of a beverage bottle reveals that a significant portion of its environmental impact comes from the production phase. Based on this insight, the company decides to switch to recycled PET materials and redesign the bottle for easier recycling, thereby reducing its overall carbon footprint and aligning with circular economy goals.
6. How does material flow analysis (MFA) support the principles of industrial ecology and the circular economy?
Answer:
Material Flow Analysis (MFA) is a systematic assessment of the flows and stocks of materials within a system, often a defined geographical area or industrial sector. MFA supports the principles of industrial ecology and the circular economy by providing a clear understanding of how materials move through industrial processes, identifying inefficiencies, and highlighting opportunities for resource optimization and waste reduction.
Support for Industrial Ecology and Circular Economy:
- Resource Tracking: Maps the movement of materials from extraction through production, consumption, and disposal, enabling a comprehensive view of resource use.
- Waste Identification: Identifies where waste is generated and lost in the system, allowing for targeted strategies to minimize waste and promote reuse or recycling.
- System Optimization: Reveals inefficiencies and bottlenecks in material flows, facilitating the redesign of processes for better resource efficiency and reduced environmental impact.
- Symbiotic Relationships: Helps in identifying potential synergies between different industries, where the waste from one process can serve as input for another, promoting symbiotic industrial ecosystems.
- Policy Development: Provides data and insights to inform policies and regulations that encourage circular practices and sustainable resource management.
- Performance Monitoring: Enables ongoing monitoring of material flows to assess the effectiveness of circular economy initiatives and industrial ecology practices.
Benefits:
- Enhanced Resource Efficiency: Promotes the optimal use of materials, reducing the need for virgin resources and minimizing waste.
- Environmental Impact Reduction: Decreases pollution and greenhouse gas emissions by improving material management and reducing resource extraction.
- Economic Savings: Lowers costs associated with raw material procurement, waste management, and disposal.
- Sustainable Growth: Supports sustainable industrial growth by ensuring that resource use is aligned with environmental and economic sustainability goals.
Example:
An MFA of a region’s manufacturing sector identifies that a significant amount of aluminum scrap is being disposed of as waste. By establishing a recycling loop where this scrap is collected and reused in new products, the region can reduce its demand for primary aluminum, lower energy consumption, and decrease greenhouse gas emissions.
7. What are the key strategies for implementing a circular economy in manufacturing industries?
Answer:
Implementing a circular economy in manufacturing industries involves adopting strategies that promote the efficient use of resources, minimize waste, and ensure that products and materials remain in circulation for as long as possible. Key strategies include:
Design for Longevity:
- Strategy: Develop products that are durable, repairable, and upgradable to extend their lifespan.
- Benefit: Reduces the need for frequent replacements, lowering resource consumption and waste generation.
Design for Disassembly:
- Strategy: Create products that can be easily disassembled at the end of their lifecycle for repair, reuse, or recycling.
- Benefit: Facilitates the recovery and recycling of materials, enhancing resource efficiency.
Resource Recovery:
- Strategy: Implement processes to reclaim materials from waste streams, such as recycling, composting, or energy recovery.
- Benefit: Transforms waste into valuable resources, reducing the need for virgin materials and minimizing landfill use.
Product-as-a-Service (PaaS):
- Strategy: Shift from selling products to providing them as services, retaining ownership and responsibility for maintenance, repair, and end-of-life management.
- Benefit: Encourages manufacturers to design products for durability and ease of maintenance, promoting reuse and extending product lifecycles.
Industrial Symbiosis:
- Strategy: Foster collaborations between different industries where the waste or by-products of one process serve as inputs for another.
- Benefit: Creates symbiotic relationships that enhance resource efficiency and reduce waste across industries.
Circular Supply Chains:
- Strategy: Develop supply chains that prioritize the use of recycled, renewable, or sustainably sourced materials.
- Benefit: Ensures that resources are sustainably managed and reduces the environmental impact of material sourcing.
Digital Technologies:
- Strategy: Utilize digital tools such as IoT, blockchain, and data analytics to track material flows, optimize resource use, and enhance transparency.
- Benefit: Improves the efficiency and effectiveness of circular economy practices through better data management and process optimization.
Closed-Loop Manufacturing:
- Strategy: Integrate processes that recycle and reuse materials within the manufacturing system, creating a continuous loop.
- Benefit: Minimizes waste and reduces the need for external inputs, enhancing sustainability.
Lean Manufacturing:
- Strategy: Adopt lean principles to eliminate waste, optimize processes, and improve overall efficiency.
- Benefit: Supports circular economy goals by reducing resource consumption and minimizing waste generation.
Collaboration and Partnerships:
- Strategy: Engage in partnerships with suppliers, customers, and other stakeholders to co-create circular solutions and share resources.
- Benefit: Enhances innovation, facilitates the sharing of best practices, and builds a supportive ecosystem for circular initiatives.
Example:
A car manufacturer implements a circular economy strategy by designing vehicles for easy disassembly, establishing a take-back program for end-of-life vehicles, and collaborating with parts suppliers to reuse and recycle components, thereby reducing waste and conserving materials.
8. How can renewable energy integration enhance the circular economy in industrial systems?
Answer:
Renewable energy integration plays a pivotal role in enhancing the circular economy within industrial systems by providing sustainable energy sources that reduce reliance on finite resources, lower greenhouse gas emissions, and support the overall sustainability of circular practices.
Enhancements through Renewable Energy Integration:
Reduced Carbon Footprint:
- Impact: Replaces fossil fuels with clean energy sources like solar, wind, hydro, and biomass, significantly lowering greenhouse gas emissions.
- Benefit: Contributes to climate change mitigation and aligns with global sustainability goals.
Energy Independence and Security:
- Impact: Reduces dependency on external energy suppliers and volatile fossil fuel markets.
- Benefit: Enhances the resilience and stability of industrial operations by ensuring a reliable and stable energy supply.
Cost Savings:
- Impact: Decreases long-term energy costs through the use of free renewable resources once initial investments are made.
- Benefit: Improves the economic viability of circular economy initiatives by reducing operational expenses.
Synergy with Waste Management:
- Impact: Biomass and waste-to-energy technologies can convert organic waste into renewable energy, closing the loop in material and energy flows.
- Benefit: Transforms waste streams into valuable energy resources, reducing landfill use and creating additional revenue streams.
Enhanced Resource Efficiency:
- Impact: Integrates renewable energy systems with energy-efficient practices, maximizing the use of available resources and minimizing waste.
- Benefit: Supports the optimization of industrial processes, leading to more sustainable and efficient operations.
Innovation and Technological Advancement:
- Impact: Drives the development and adoption of new renewable energy technologies and smart energy management systems.
- Benefit: Promotes continuous improvement and innovation within industrial sectors, enhancing their sustainability and competitiveness.
Regulatory Compliance and Incentives:
- Impact: Helps industries comply with environmental regulations and take advantage of government incentives for renewable energy adoption.
- Benefit: Reduces financial barriers and accelerates the implementation of circular economy practices.
Example:
An industrial park incorporates solar panels and a biomass energy system to power its manufacturing facilities. Excess energy generated is fed back into the grid or used to power additional processes, creating a sustainable and closed-loop energy system that supports circular economy principles by minimizing waste and maximizing resource use.
9. Discuss the importance of product design in achieving a circular economy and how engineers can incorporate circular principles into their designs.
Answer:
Product design is fundamental to achieving a circular economy as it determines how products are made, used, and disposed of, directly influencing their environmental impact and lifecycle. Engineers play a crucial role in incorporating circular principles into product designs to ensure sustainability, resource efficiency, and waste minimization.
Importance of Product Design:
- Lifecycle Impact: Design choices affect the entire lifecycle of a product, from resource extraction to end-of-life disposal.
- Resource Efficiency: Optimizes the use of materials and energy, reducing the environmental footprint.
- Waste Minimization: Facilitates reuse, repair, refurbishment, and recycling, minimizing waste generation.
- Economic Viability: Enhances the economic sustainability of products by extending their lifespan and reducing costs associated with waste management.
- Consumer Satisfaction: Meets the growing demand for sustainable and eco-friendly products, improving market competitiveness.
Incorporating Circular Principles into Designs:
Design for Durability:
- Strategy: Create products that are robust, reliable, and long-lasting.
- Benefit: Extends the product’s useful life, reducing the need for frequent replacements.
Design for Repairability:
- Strategy: Ensure that products can be easily repaired and maintained.
- Benefit: Facilitates the fixing of damaged components, extending the product’s lifespan and reducing waste.
Design for Disassembly:
- Strategy: Develop products that can be easily taken apart at the end of their lifecycle.
- Benefit: Simplifies the recovery and recycling of materials, enhancing resource efficiency.
Modular Design:
- Strategy: Use modular components that can be replaced or upgraded without discarding the entire product.
- Benefit: Allows for easy customization, upgrades, and repairs, promoting longevity and adaptability.
Use of Recyclable and Sustainable Materials:
- Strategy: Select materials that are easily recyclable, renewable, or have low environmental impact.
- Benefit: Ensures that materials can be recovered and reused, minimizing environmental footprint.
Minimalist Design:
- Strategy: Simplify product designs to use fewer materials and reduce complexity.
- Benefit: Decreases resource use and facilitates easier disassembly and recycling.
Biodegradable and Compostable Designs:
- Strategy: Incorporate materials that can safely decompose in natural environments.
- Benefit: Reduces waste and the environmental impact of disposed products.
Embedded Reuse Opportunities:
- Strategy: Design products with built-in options for reuse or repurposing in different applications.
- Benefit: Extends the product’s usefulness and reduces the need for new resources.
Energy-Efficient Manufacturing:
- Strategy: Optimize manufacturing processes to minimize energy consumption and emissions.
- Benefit: Enhances the overall sustainability of the product from production to end-of-life.
Smart and Connected Features:
- Strategy: Incorporate digital technologies that monitor product performance and facilitate maintenance and upgrades.
- Benefit: Enhances product longevity and enables data-driven sustainability improvements.
Example:
A smartphone manufacturer designs its devices with modular components that can be easily replaced or upgraded, uses recyclable materials, and ensures that the product can be disassembled without damaging the components. This approach facilitates repair, reuse, and recycling, aligning with circular economy principles and reducing electronic waste.
Conclusion: Product design is a critical element in achieving a circular economy, as it influences the sustainability and lifecycle of products. By incorporating circular principles such as durability, repairability, disassembly, modularity, and the use of sustainable materials, engineers can create products that minimize environmental impact, optimize resource use, and support the transition to a circular economy. Thoughtful design not only enhances product longevity and reduces waste but also meets the growing consumer demand for sustainable products, driving economic and environmental benefits.
10. How can data interoperability standards facilitate the integration of diverse environmental datasets, and what are the benefits of achieving seamless data interoperability in environmental engineering projects?
Answer:
Data interoperability standards are frameworks and protocols that enable different data systems and formats to communicate, share, and utilize data seamlessly. In environmental engineering projects, achieving data interoperability is essential for integrating diverse datasets from various sources, enhancing the efficiency and effectiveness of data analysis and decision-making.
Facilitation of Integration:
Standardized Data Formats:
- Function: Establish common data structures and formats (e.g., JSON, XML, CSV) that allow different systems to interpret and use data consistently.
- Benefit: Ensures compatibility between datasets from different sensors, platforms, and organizations, enabling smooth data exchange and aggregation.
Common Metadata Standards:
- Function: Define standardized metadata schemas that describe the context, quality, and structure of datasets.
- Benefit: Enhances data discoverability, usability, and comparability by providing consistent descriptions of data sources and attributes.
APIs and Data Sharing Protocols:
- Function: Develop application programming interfaces (APIs) and protocols (e.g., OGC standards) that facilitate automated data sharing and integration.
- Benefit: Enables real-time data exchange and interoperability between disparate systems, supporting dynamic and collaborative data usage.
Semantic Standards and Ontologies:
- Function: Utilize standardized vocabularies and ontologies to ensure that data meanings are consistently understood across different systems.
- Benefit: Prevents misinterpretation of data by aligning terminologies and concepts, enabling accurate data integration and analysis.
Data Governance Frameworks:
- Function: Implement policies and practices that ensure data quality, security, and compliance with interoperability standards.
- Benefit: Promotes trust and reliability in integrated datasets, ensuring that data can be effectively used for decision-making.
Benefits of Seamless Data Interoperability:
Enhanced Data Accessibility and Sharing:
- Benefit: Facilitates easy access to a wide range of datasets, promoting collaboration among environmental engineers, researchers, and stakeholders.
- Impact: Accelerates the development of comprehensive environmental assessments and solutions by leveraging diverse data sources.
Improved Data Quality and Consistency:
- Benefit: Ensures that integrated datasets are consistent, accurate, and reliable, reducing errors and enhancing the validity of analyses.
- Impact: Leads to more robust and credible environmental engineering outcomes, supporting effective decision-making and policy development.
Increased Efficiency and Reduced Redundancy:
- Benefit: Eliminates the need for manual data conversion and duplication, saving time and resources.
- Impact: Streamlines data workflows, allowing engineers to focus on analysis and application rather than data management.
Facilitated Comprehensive Analysis:
- Benefit: Enables the combination of diverse datasets (e.g., air quality, water quality, land use) for holistic environmental analysis.
- Impact: Provides a more complete understanding of environmental systems, supporting integrated and multifaceted engineering solutions.
Support for Advanced Technologies:
- Benefit: Enhances the effectiveness of machine learning, artificial intelligence, and big data analytics by providing well-integrated and standardized datasets.
- Impact: Unlocks new possibilities for predictive modeling, trend analysis, and innovative environmental engineering applications.
Scalability and Adaptability:
- Benefit: Allows environmental monitoring systems to scale and adapt by easily incorporating new data sources and technologies.
- Impact: Ensures that environmental engineering projects remain flexible and can evolve with emerging data needs and technological advancements.
Example:
An environmental engineering project aiming to assess urban air quality can integrate data from satellite remote sensing, ground-based sensor networks, and traffic monitoring systems using standardized data formats and metadata. This seamless integration allows for comprehensive analysis of pollution sources, dispersion patterns, and the effectiveness of mitigation measures.
Conclusion: Data interoperability standards are crucial for integrating diverse environmental datasets, enabling seamless data sharing, enhancing data quality, and supporting comprehensive analysis in environmental engineering projects. Achieving data interoperability fosters collaboration, increases efficiency, and facilitates the use of advanced technologies, ultimately leading to more effective and informed environmental management and engineering solutions.
Industrial Ecology and Circular Economy: Thought-Provoking Questions with Detailed Answers:
1. How can the principles of industrial ecology be applied to develop sustainable urban infrastructure, and what are the potential benefits and challenges of such applications?
Answer:
The principles of industrial ecology can be applied to develop sustainable urban infrastructure by creating systems that emulate natural ecosystems, where waste from one process becomes input for another. This approach integrates various urban sectors—such as energy, water, transportation, and waste management—to optimize resource use and minimize environmental impact.
Application:
- Energy Systems: Implementing district energy systems that utilize waste heat from industrial processes to heat residential and commercial buildings.
- Water Management: Developing integrated water management systems where treated wastewater is reused for irrigation and industrial processes.
- Waste-to-Resource Programs: Establishing facilities that convert municipal waste into raw materials for manufacturing, reducing landfill use and conserving natural resources.
- Transportation Synergies: Coordinating public transportation with renewable energy sources to reduce carbon emissions and enhance energy efficiency.
Benefits:
- Resource Efficiency: Maximizes the use of available resources, reducing the need for virgin materials and conserving natural resources.
- Environmental Impact Reduction: Lowers greenhouse gas emissions, pollution, and waste generation by optimizing resource flows and minimizing waste.
- Economic Savings: Reduces operational costs through the efficient use of resources and the creation of new revenue streams from waste-to-resource initiatives.
- Resilience and Adaptability: Enhances the resilience of urban infrastructure by diversifying resource sources and creating flexible systems that can adapt to changing conditions.
Challenges:
- System Complexity: Coordinating multiple sectors and processes requires sophisticated planning and management, which can be complex and resource-intensive.
- Initial Investment Costs: Establishing integrated systems often requires significant upfront capital, which may be a barrier for municipalities and businesses.
- Technological Integration: Integrating diverse technologies and ensuring their compatibility can be technically challenging.
- Stakeholder Collaboration: Achieving effective collaboration among various stakeholders, including government agencies, businesses, and communities, can be difficult due to differing priorities and interests.
- Regulatory Hurdles: Navigating and harmonizing regulations across different sectors can impede the implementation of industrial ecology practices.
Example:
A city implements an industrial ecology-based district energy system that captures waste heat from a nearby manufacturing plant to provide heating for residential buildings. This reduces the city’s overall energy consumption, lowers emissions, and provides cost savings for residents. However, the project faces challenges in coordinating between the manufacturing plant, energy providers, and residential developers, as well as securing the necessary funding and regulatory approvals.
2. In what ways can the circular economy transform traditional manufacturing processes, and what role do engineers play in facilitating this transformation?
Answer:
The circular economy can transform traditional manufacturing processes by shifting from a linear ‘take-make-dispose’ model to a closed-loop system that emphasizes resource efficiency, waste minimization, and the continual use of materials. This transformation involves redesigning products and processes to support reuse, recycling, and remanufacturing, thereby enhancing sustainability and reducing environmental impact.
Transformations in Manufacturing Processes:
- Material Efficiency: Reducing material usage through design optimization and selecting sustainable materials.
- Waste Minimization: Implementing processes that generate minimal waste and ensuring that any waste produced is reused or recycled.
- Product Lifecycle Extension: Designing products for longevity, ease of maintenance, and upgradeability to extend their usable life.
- Resource Recovery: Establishing systems for recovering and reusing materials from end-of-life products, creating a closed-loop supply chain.
- Energy Efficiency: Enhancing energy efficiency in manufacturing processes to reduce energy consumption and emissions.
Role of Engineers:
- Innovative Design: Developing new product designs that prioritize durability, repairability, and recyclability, ensuring that products can be easily disassembled and materials reused.
- Process Optimization: Redesigning manufacturing processes to minimize resource use, reduce waste, and incorporate recycled materials, enhancing overall efficiency and sustainability.
- Technology Development: Creating and implementing technologies that facilitate material recovery, recycling, and the efficient use of resources, such as advanced recycling techniques and energy-efficient machinery.
- Systems Integration: Coordinating different components of the manufacturing system to create symbiotic relationships where waste from one process becomes input for another, mimicking natural ecosystems.
- Lifecycle Analysis: Conducting lifecycle assessments to evaluate the environmental impact of products and processes, guiding decisions to enhance sustainability.
- Collaboration and Leadership: Leading interdisciplinary teams and collaborating with stakeholders across the supply chain to implement circular economy practices effectively.
Benefits:
- Environmental Sustainability: Reduces the environmental footprint of manufacturing by conserving resources, lowering emissions, and minimizing waste.
- Economic Efficiency: Lowers operational costs through efficient resource use and waste reduction, and creates new revenue streams from recycled materials and remanufactured products.
- Competitive Advantage: Positions companies as leaders in sustainability, attracting environmentally conscious consumers and investors.
- Resilience: Enhances the resilience of manufacturing operations by reducing dependency on raw material extraction and mitigating risks associated with resource scarcity.
Example:
An automotive manufacturer redesigns its vehicles to use modular components that can be easily replaced or upgraded. Engineers develop processes for collecting and recycling used parts, integrating recycled materials into new vehicles. This circular approach reduces material costs, lowers environmental impact, and extends the lifecycle of vehicle components, while also positioning the company as a sustainable leader in the automotive industry.
3. What are the key indicators used to measure the success of circular economy initiatives in industrial ecology, and how can these indicators guide future improvements?
Answer:
Key indicators used to measure the success of circular economy initiatives in industrial ecology focus on assessing resource efficiency, waste reduction, economic performance, and environmental impact. These indicators provide quantitative metrics that help evaluate the effectiveness of circular practices and identify areas for improvement.
Key Indicators:
Material Circularity Indicator (MCI):
- Description: Measures the extent to which materials are reused, recycled, or repurposed within a system.
- Purpose: Evaluates the circularity of material flows and identifies opportunities to increase resource reuse.
Resource Productivity:
- Description: Assesses the economic value generated per unit of resource consumed.
- Purpose: Indicates the efficiency of resource use and the economic benefits of circular practices.
Waste Reduction Rate:
- Description: Calculates the percentage reduction in waste generation over time.
- Purpose: Tracks the effectiveness of waste minimization strategies and identifies areas needing improvement.
Recycling Rate:
- Description: Measures the proportion of waste materials that are recycled compared to the total waste generated.
- Purpose: Evaluates the success of recycling programs and the efficiency of material recovery processes.
Energy Efficiency:
- Description: Assesses the amount of energy used per unit of production or service.
- Purpose: Indicates improvements in energy use and the effectiveness of energy-saving measures.
Carbon Footprint:
- Description: Quantifies the total greenhouse gas emissions associated with a product, process, or system.
- Purpose: Measures the environmental impact and effectiveness of carbon reduction initiatives.
Water Footprint:
- Description: Measures the total volume of freshwater used to produce goods and services.
- Purpose: Assesses water use efficiency and identifies opportunities for water conservation.
Product Lifecycle Extension:
- Description: Tracks the average lifespan of products and the extent to which they are reused, repaired, or refurbished.
- Purpose: Evaluates the success of strategies aimed at extending product lifecycles and reducing the need for new resources.
Circular Supply Chain Index:
- Description: Measures the degree to which supply chains incorporate circular principles such as material reuse and recycling.
- Purpose: Assesses the integration of circular practices across the entire supply chain and identifies collaboration opportunities.
Economic Indicators:
- Description: Includes metrics such as cost savings, return on investment (ROI), and revenue from recycled materials.
- Purpose: Evaluates the financial performance and economic benefits of circular economy initiatives.
Guiding Future Improvements:
- Benchmarking: Compare indicators against industry standards or best practices to identify performance gaps and set improvement targets.
- Data-Driven Decisions: Use indicator trends to inform strategic decisions, allocate resources effectively, and prioritize initiatives that yield the highest benefits.
- Continuous Monitoring: Regularly track and review indicators to ensure that circular economy practices are on track and to make timely adjustments as needed.
- Stakeholder Engagement: Share indicator results with stakeholders to demonstrate progress, build trust, and encourage continued collaboration and support for circular initiatives.
- Innovation Focus: Identify areas where indicators show underperformance and invest in research and development to innovate and implement more effective circular solutions.
Example:
A manufacturing company monitors its Material Circularity Indicator (MCI) and notices a declining trend in material reuse. In response, engineers investigate the underlying causes, such as inefficiencies in the collection process, and implement improvements like automated sorting technologies and enhanced recycling partnerships. This leads to an increase in the MCI, demonstrating improved circularity and guiding further enhancements in material management practices.
4. How can the integration of digital technologies, such as IoT and blockchain, enhance the implementation of circular economy practices in industrial ecology?
Answer:
The integration of digital technologies like the Internet of Things (IoT) and blockchain can significantly enhance the implementation of circular economy practices in industrial ecology by providing real-time data, improving transparency, facilitating efficient resource management, and enabling secure and traceable transactions.
Enhancements through Digital Technologies:
Internet of Things (IoT):
- Real-Time Monitoring: IoT devices can monitor the flow of materials, energy usage, and environmental conditions in real-time, providing valuable data for optimizing circular processes.
- Predictive Maintenance: Sensors can predict equipment failures before they occur, reducing downtime and extending the lifespan of machinery.
- Resource Tracking: IoT enables precise tracking of materials throughout their lifecycle, ensuring that resources are reused, recycled, or repurposed effectively.
- Automation and Efficiency: Automates processes such as sorting, recycling, and resource allocation, increasing operational efficiency and reducing human error.
Blockchain:
- Transparency and Traceability: Blockchain provides an immutable ledger that records every transaction and movement of materials, ensuring transparency and traceability in the supply chain.
- Smart Contracts: Automates agreements and transactions, facilitating efficient and secure exchanges of resources without intermediaries.
- Incentivizing Circular Practices: Implements token-based systems that reward companies and individuals for participating in circular activities, such as recycling or returning used products.
- Reducing Fraud: Enhances the integrity of circular systems by preventing fraud and ensuring that recycled materials are properly accounted for and reused.
Benefits of Integration:
- Enhanced Data Accuracy: Provides precise and reliable data for making informed decisions about resource management and circular practices.
- Improved Collaboration: Facilitates seamless information sharing among stakeholders, promoting collaboration and the creation of symbiotic industrial relationships.
- Increased Efficiency: Streamlines operations by automating processes and optimizing resource flows, reducing costs and minimizing waste.
- Greater Accountability: Ensures that all participants in the circular economy are accountable for their actions, enhancing trust and cooperation.
- Innovation and Scalability: Supports the development of innovative circular solutions and enables scalable implementations across different industries and regions.
Example:
A recycling company uses IoT sensors to track the collection and processing of materials, monitoring the quantity and quality of recyclables in real-time. This data is recorded on a blockchain platform, providing transparent and traceable records of material flows. Smart contracts automate payments to collectors based on the volume and quality of recyclables delivered, incentivizing higher participation rates and ensuring that materials are efficiently reused or recycled.
Conclusion: The integration of digital technologies like IoT and blockchain plays a crucial role in advancing circular economy practices within industrial ecology. By enhancing real-time monitoring, transparency, traceability, and automation, these technologies enable more efficient and effective resource management, foster collaboration, and support the secure and scalable implementation of circular initiatives. Leveraging digital innovations can significantly accelerate the transition to a circular economy, driving sustainability and resilience in industrial systems.
5. What role do policies and regulations play in promoting industrial ecology and the circular economy, and how can engineers advocate for effective policy frameworks?
Answer:
Policies and regulations play a critical role in promoting industrial ecology and the circular economy by providing the legal framework, incentives, and guidelines necessary to drive sustainable practices, resource efficiency, and waste minimization. These policies can mandate sustainable design standards, incentivize circular business models, and create market conditions that support the transition from a linear to a circular economy.
Roles of Policies and Regulations:
Setting Standards and Guidelines:
- Function: Establish minimum requirements for sustainability, resource use, and waste management in industrial processes and product designs.
- Impact: Ensures that all industries adhere to baseline sustainability practices, leveling the playing field and preventing free-riding.
Incentivizing Circular Practices:
- Function: Provide financial incentives such as tax credits, grants, and subsidies for businesses that adopt circular economy practices.
- Impact: Reduces the financial barriers to implementing circular initiatives, encouraging more companies to participate.
Mandating Waste Reduction and Recycling:
- Function: Require industries to minimize waste generation, recycle materials, and manage waste responsibly.
- Impact: Drives waste reduction efforts and promotes the establishment of recycling and resource recovery systems.
Promoting Innovation and Research:
- Function: Fund research and development projects focused on circular economy technologies and practices.
- Impact: Stimulates innovation and the development of new solutions that support circularity and sustainability.
Facilitating Collaboration and Partnerships:
- Function: Encourage collaboration between different sectors, industries, and stakeholders to develop integrated circular solutions.
- Impact: Promotes the sharing of knowledge, resources, and best practices, fostering a collaborative approach to circular economy implementation.
Enforcing Compliance and Accountability:
- Function: Implement monitoring, reporting, and enforcement mechanisms to ensure compliance with environmental regulations and circular economy standards.
- Impact: Holds industries accountable for their environmental performance, ensuring that policies translate into tangible sustainability outcomes.
Engineers’ Role in Advocating for Effective Policy Frameworks:
Engaging in Policy Development:
- Action: Participate in consultations, provide expert testimony, and contribute to the formulation of policies that support industrial ecology and the circular economy.
- Benefit: Ensures that policies are technically sound, practical, and effectively address the challenges and opportunities of circular practices.
Providing Evidence-Based Insights:
- Action: Use data, case studies, and research to demonstrate the benefits and feasibility of circular economy initiatives.
- Benefit: Strengthens the case for supportive policies by showcasing real-world examples and quantifiable benefits.
Collaborating with Stakeholders:
- Action: Work with industry associations, government agencies, non-profits, and other stakeholders to advocate for policies that facilitate circular economy practices.
- Benefit: Builds a unified voice and collective advocacy efforts, increasing the likelihood of policy adoption and support.
Educating and Raising Awareness:
- Action: Conduct workshops, seminars, and informational campaigns to educate policymakers and the public about the importance and benefits of industrial ecology and the circular economy.
- Benefit: Enhances understanding and support for circular economy policies, fostering a culture of sustainability.
Demonstrating Best Practices:
- Action: Lead by example by implementing circular economy practices in engineering projects and showcasing their success.
- Benefit: Provides tangible proof of the effectiveness of circular practices, inspiring policymakers to adopt supportive frameworks.
Advocating for Incentives and Support Mechanisms:
- Action: Push for policies that provide financial and technical support to businesses transitioning to circular models.
- Benefit: Facilitates the adoption of circular practices by reducing costs and providing the necessary resources for implementation.
Example:
Engineers working in the renewable energy sector collaborate with government agencies to develop incentives for using recycled materials in solar panel manufacturing. They present data showing the environmental and economic benefits of material recycling, advocate for supportive policies, and help design standards that promote the use of sustainable materials. This collaborative effort leads to the adoption of new regulations that encourage the circular use of resources in the renewable energy industry.
Conclusion: Policies and regulations are essential for fostering industrial ecology and the circular economy by providing the necessary framework, incentives, and standards to drive sustainable practices. Engineers play a pivotal role in advocating for effective policy frameworks by engaging in policy development, providing evidence-based insights, collaborating with stakeholders, educating and raising awareness, demonstrating best practices, and advocating for supportive incentives. Through these efforts, engineers can help shape policies that facilitate the transition to a circular economy, promoting sustainability and resilience in industrial systems.
6. How can collaboration between different industries enhance the effectiveness of circular economy initiatives in industrial ecology?
Answer:
Collaboration between different industries can significantly enhance the effectiveness of circular economy initiatives in industrial ecology by creating synergies, optimizing resource flows, and fostering innovation. By working together, industries can transform waste streams into valuable inputs, share resources and technologies, and develop integrated systems that support circularity across the entire value chain.
Enhancements Through Collaboration:
Resource Synergy:
- Benefit: One industry’s waste can serve as another’s raw material, creating a symbiotic relationship that reduces waste and conserves resources.
- Example: A food processing plant’s organic waste is used by a bioenergy company to produce biogas, providing a sustainable energy source while minimizing waste disposal.
Shared Infrastructure:
- Benefit: Collaborative use of facilities and infrastructure, such as recycling centers, energy grids, and transportation networks, can reduce costs and improve efficiency.
- Example: Multiple manufacturing firms share a centralized recycling facility to process and reuse materials, lowering individual costs and enhancing material recovery rates.
Innovation and Knowledge Sharing:
- Benefit: Sharing expertise, technologies, and best practices across industries fosters innovation and accelerates the development of circular solutions.
- Example: Engineers from the automotive and electronics industries collaborate to develop standardized components that are easier to repair and recycle, promoting circularity in both sectors.
Economies of Scale:
- Benefit: Joint initiatives can achieve economies of scale, making circular practices more economically viable and attractive to businesses.
- Example: A consortium of textile manufacturers invests in a large-scale textile recycling facility, reducing the cost per unit of recycled material and increasing the availability of recycled fibers.
Systemic Approach:
- Benefit: Collaboration enables a holistic view of resource flows and environmental impacts, supporting the design of integrated and sustainable industrial ecosystems.
- Example: Industries within an industrial park coordinate their waste and energy flows to create a closed-loop system, where excess heat from one process is used by another and waste materials are repurposed internally.
Market Development:
- Benefit: Joint efforts can help create new markets for recycled materials and circular products, increasing demand and incentivizing circular practices.
- Example: Industries collaborate to develop standards and certifications for recycled materials, enhancing consumer trust and market acceptance.
Strategies for Effective Collaboration:
Establishing Partnerships:
- Strategy: Form strategic alliances and partnerships between industries with complementary resources and capabilities.
- Benefit: Facilitates resource sharing, joint investments, and coordinated efforts towards common circular economy goals.
Creating Industrial Symbiosis Networks:
- Strategy: Develop networks that connect different industries to identify and exploit mutual waste and resource exchange opportunities.
- Benefit: Enhances resource efficiency and creates sustainable industrial ecosystems through shared waste streams and resource inputs.
Joint Research and Development:
- Strategy: Collaborate on R&D projects to innovate circular solutions, such as advanced recycling technologies and sustainable product designs.
- Benefit: Accelerates the development and adoption of new circular technologies and practices through shared knowledge and resources.
Standardization and Harmonization:
- Strategy: Work together to develop standardized processes, materials, and technologies that support circularity across industries.
- Benefit: Promotes compatibility and interoperability, making it easier to integrate circular practices and scale them across different sectors.
Shared Education and Training:
- Strategy: Provide joint training programs and educational initiatives to build a workforce skilled in circular economy practices.
- Benefit: Enhances the capacity of industries to implement circular initiatives effectively through shared knowledge and expertise.
Example:
A steel manufacturer collaborates with a construction company to use recycled steel from demolished buildings in new construction projects. This partnership not only reduces the demand for virgin steel but also minimizes construction waste, supporting both companies’ sustainability goals and enhancing the overall circularity of the construction industry.
Conclusion: Collaboration between different industries is a key driver for the success of circular economy initiatives in industrial ecology. By creating synergies, sharing resources and expertise, and developing integrated systems, industries can optimize resource use, reduce waste, and foster innovation. Effective collaboration leads to enhanced resource efficiency, economic savings, environmental benefits, and the development of resilient and sustainable industrial ecosystems. Engineers play a crucial role in facilitating these collaborations by identifying opportunities, designing integrated systems, and leading interdisciplinary teams towards common circular economy objectives.
7. What are the economic and environmental benefits of adopting circular economy practices in industrial ecosystems?
Answer:
Adopting circular economy practices in industrial ecosystems offers significant economic and environmental benefits by promoting resource efficiency, reducing waste, and fostering sustainable growth. These benefits contribute to the resilience and competitiveness of industries while minimizing their environmental footprint.
Economic Benefits:
Cost Savings:
- Description: Reducing material and energy consumption lowers operational costs.
- Impact: Saves money on raw materials, energy bills, and waste disposal fees.
Revenue Generation:
- Description: Creating new revenue streams from recycled materials, remanufactured products, and waste-to-energy initiatives.
- Impact: Increases profitability and diversifies income sources.
Job Creation:
- Description: Developing recycling facilities, remanufacturing plants, and maintenance services creates new employment opportunities.
- Impact: Boosts local economies and supports community development.
Market Competitiveness:
- Description: Offering sustainable products and services can differentiate businesses in the marketplace.
- Impact: Attracts environmentally conscious consumers and investors, enhancing market share and brand reputation.
Risk Mitigation:
- Description: Reducing dependency on finite resources and mitigating exposure to volatile raw material prices.
- Impact: Enhances business resilience and stability in the face of resource scarcity and market fluctuations.
Compliance and Incentives:
- Description: Meeting regulatory requirements and accessing financial incentives for sustainable practices.
- Impact: Avoids fines and penalties, while benefiting from subsidies, tax credits, and grants.
Environmental Benefits:
Resource Conservation:
- Description: Maximizes the use of existing materials, reducing the need for virgin resource extraction.
- Impact: Preserves natural resources and reduces environmental degradation associated with mining, logging, and drilling.
Waste Reduction:
- Description: Minimizes waste generation through reuse, recycling, and efficient resource management.
- Impact: Decreases landfill use, reduces pollution, and mitigates the environmental impact of waste disposal.
Lower Greenhouse Gas Emissions:
- Description: Reducing resource extraction, manufacturing, and transportation lowers carbon emissions.
- Impact: Contributes to climate change mitigation and improves air quality.
Ecosystem Protection:
- Description: Preserves biodiversity and natural habitats by reducing the pressure on ecosystems from resource extraction and waste.
- Impact: Maintains ecosystem services and enhances environmental resilience.
Energy Efficiency:
- Description: Optimizing energy use in processes and incorporating renewable energy sources.
- Impact: Reduces overall energy consumption and reliance on fossil fuels, lowering carbon emissions and energy costs.
Sustainable Product Lifecycle:
- Description: Designing products for durability, repairability, and recyclability ensures sustainable use throughout their lifecycle.
- Impact: Extends product lifespans, reduces the need for new products, and minimizes environmental impact.
Example:
A textile manufacturer adopts circular economy practices by using recycled fibers, implementing water-efficient dyeing processes, and establishing a take-back program for used garments. Economically, the company reduces material costs and creates a new revenue stream from recycled textiles. Environmentally, it conserves water, reduces textile waste, and lowers its carbon footprint, contributing to sustainability and enhancing its brand reputation.
Conclusion: Adopting circular economy practices in industrial ecosystems yields substantial economic and environmental benefits. Economically, it reduces costs, generates new revenues, enhances market competitiveness, and creates jobs, while also mitigating risks and ensuring regulatory compliance. Environmentally, it conserves resources, reduces waste, lowers greenhouse gas emissions, protects ecosystems, and promotes energy efficiency. Together, these benefits contribute to the sustainability and resilience of industries, supporting long-term economic growth and environmental stewardship.
8. How can engineers utilize lifecycle assessment (LCA) to improve the sustainability of products within an industrial ecology framework?
Answer:
Engineers can utilize Lifecycle Assessment (LCA) as a comprehensive tool to evaluate and enhance the sustainability of products within an industrial ecology framework. By assessing the environmental impacts of products from cradle to grave, engineers can identify opportunities for improvement, optimize resource use, and integrate circular economy principles into product design and manufacturing processes.
Utilization of LCA:
Identifying Environmental Hotspots:
- Action: Conduct LCA to pinpoint stages in the product lifecycle with the highest environmental impacts, such as raw material extraction, manufacturing, transportation, use, and disposal.
- Benefit: Focuses efforts on areas where sustainability improvements can have the most significant impact.
Optimizing Material Selection:
- Action: Use LCA to compare the environmental impacts of different materials and select those with lower embodied energy, reduced emissions, and sustainable sourcing.
- Benefit: Minimizes the environmental footprint of products by choosing more sustainable materials.
Enhancing Design for Sustainability:
- Action: Incorporate LCA findings into the design process to create products that are easier to repair, upgrade, and recycle, thereby extending their lifecycle and reducing waste.
- Benefit: Promotes circularity by ensuring that products are designed with their entire lifecycle in mind.
Improving Manufacturing Processes:
- Action: Analyze the environmental impacts of manufacturing processes and identify opportunities to reduce energy consumption, water use, and emissions.
- Benefit: Enhances resource efficiency and lowers the environmental impact of production activities.
Facilitating Resource Recovery:
- Action: Design products and systems that facilitate the recovery and reuse of materials at the end of their lifecycle, supported by LCA insights on material flows.
- Benefit: Supports the establishment of closed-loop systems, reducing the need for virgin materials and minimizing waste.
Informing Policy and Strategy:
- Action: Use LCA data to inform corporate sustainability strategies, policy development, and regulatory compliance efforts.
- Benefit: Ensures that sustainability initiatives are data-driven and aligned with environmental goals and regulatory requirements.
Communicating Sustainability Performance:
- Action: Present LCA results to stakeholders, including customers, investors, and regulatory bodies, to demonstrate the environmental performance of products.
- Benefit: Enhances transparency, builds trust, and supports marketing efforts focused on sustainability.
Benefits within Industrial Ecology Framework:
- Holistic View: Provides a comprehensive understanding of environmental impacts across the entire product lifecycle, enabling systemic improvements.
- Data-Driven Decisions: Empowers engineers to make informed decisions based on quantitative data, enhancing the sustainability of products and processes.
- Continuous Improvement: Facilitates ongoing evaluation and refinement of products and systems to achieve higher levels of sustainability and circularity.
- Integration of Circular Principles: Supports the integration of reuse, recycling, and resource recovery into product design and industrial processes, aligning with circular economy goals.
Example:
An electronics company conducts an LCA on its smartphones to evaluate the environmental impacts of different components and materials. The assessment reveals that the battery and display have the highest energy consumption and carbon emissions. Based on these insights, engineers redesign the battery to use less toxic materials, improve energy efficiency, and implement a recycling program for used displays. These changes reduce the overall environmental footprint of the smartphones and support the company’s commitment to sustainability within an industrial ecology framework.
Conclusion: Lifecycle Assessment (LCA) is an invaluable tool for engineers seeking to improve the sustainability of products within an industrial ecology framework. By providing a detailed analysis of environmental impacts across the entire product lifecycle, LCA enables engineers to make informed decisions, optimize resource use, and integrate circular economy principles into product design and manufacturing. This leads to the development of more sustainable, efficient, and resilient products that contribute to the overall goals of industrial ecology and the circular economy.
9. What are the key components of an effective circular supply chain, and how can engineers design supply chains to support circular economy principles?
Answer:
An effective circular supply chain is designed to maximize resource efficiency, minimize waste, and ensure the continuous use and reuse of materials and products. Key components of such a supply chain include sustainable sourcing, efficient production processes, product longevity, reverse logistics, and collaboration among stakeholders. Engineers play a crucial role in designing supply chains that align with circular economy principles by integrating these components into the supply chain strategy and operations.
Key Components:
Sustainable Sourcing:
- Description: Procuring materials that are renewable, recycled, or sustainably managed.
- Importance: Ensures that the inputs to the supply chain have minimal environmental impact and support circular practices.
Efficient Production Processes:
- Description: Implementing manufacturing processes that optimize resource use, reduce energy consumption, and minimize waste.
- Importance: Enhances resource efficiency and lowers the environmental footprint of production activities.
Product Longevity and Durability:
- Description: Designing products to be durable, easy to repair, and upgradable to extend their useful life.
- Importance: Reduces the frequency of product replacement, conserving resources and minimizing waste generation.
Modular Design:
- Description: Creating products with interchangeable and easily replaceable components.
- Importance: Facilitates repair, upgrade, and recycling, supporting the continuous use of materials.
Reverse Logistics:
- Description: Establishing systems for collecting, sorting, and processing used products for reuse, refurbishment, or recycling.
- Importance: Closes the loop by ensuring that materials are recovered and reintegrated into the supply chain, reducing the need for virgin resources.
Recycling and Resource Recovery:
- Description: Implementing processes to recover materials from waste streams and reintegrate them into the production cycle.
- Importance: Enhances resource efficiency and reduces reliance on finite resources.
Collaboration and Partnerships:
- Description: Building strong relationships with suppliers, customers, and other stakeholders to facilitate circular practices.
- Importance: Promotes shared goals, resource sharing, and collaborative problem-solving, enhancing the overall effectiveness of the circular supply chain.
Digital Technologies:
- Description: Utilizing technologies such as IoT, blockchain, and data analytics to track material flows, optimize supply chain operations, and ensure transparency.
- Importance: Enhances visibility, efficiency, and traceability, supporting effective circular practices.
Product-as-a-Service (PaaS) Models:
- Description: Shifting from selling products to providing them as services, retaining ownership and responsibility for maintenance, repair, and end-of-life management.
- Importance: Encourages manufacturers to design for durability and ease of maintenance, promoting the reuse and longevity of products.
Performance Monitoring and Continuous Improvement:
- Description: Regularly assessing supply chain performance against circular economy metrics and implementing improvements based on findings.
- Importance: Ensures that the supply chain remains aligned with circular principles and adapts to changing conditions and opportunities.
Designing Circular Supply Chains:
Integrate Circular Principles into Supply Chain Strategy:
- Action: Embed circular economy objectives into the overall supply chain strategy, ensuring alignment with sustainability goals.
- Benefit: Creates a clear framework for implementing circular practices across the supply chain.
Collaborate with Stakeholders:
- Action: Engage with suppliers, customers, and partners to develop shared goals and collaborative initiatives that support circularity.
- Benefit: Fosters a collaborative environment where circular practices can thrive through shared resources and knowledge.
Implement Modular and Standardized Design:
- Action: Design products with standardized, interchangeable components to facilitate repair, upgrade, and recycling.
- Benefit: Enhances product longevity and simplifies reverse logistics and resource recovery.
Develop Robust Reverse Logistics Systems:
- Action: Establish efficient systems for collecting, sorting, and processing used products, ensuring that materials are effectively reintegrated into the supply chain.
- Benefit: Closes the material loop, reducing waste and conserving resources.
Leverage Digital Technologies:
- Action: Use IoT for real-time tracking of materials, blockchain for transparent record-keeping, and data analytics for optimizing supply chain operations.
- Benefit: Enhances visibility, efficiency, and traceability, supporting effective circular practices.
Adopt Product-as-a-Service Models:
- Action: Shift from ownership-based models to service-based models, retaining responsibility for product maintenance and end-of-life management.
- Benefit: Incentivizes manufacturers to design durable, repairable, and recyclable products, promoting circularity.
Focus on Lifecycle Thinking:
- Action: Consider the entire lifecycle of products and materials in supply chain planning and decision-making.
- Benefit: Identifies opportunities for sustainability improvements and circular practices at every stage of the lifecycle.
Invest in Recycling and Resource Recovery Infrastructure:
- Action: Develop or partner with facilities that can efficiently recycle and recover materials from waste streams.
- Benefit: Ensures that materials are effectively reused and reintegrated into the supply chain, reducing the need for virgin resources.
Example:
A furniture manufacturer designs its products with modular components that can be easily disassembled and reassembled. They establish a reverse logistics system where customers can return old furniture for refurbishment or recycling. By collaborating with recycling facilities and using digital tracking systems, the manufacturer ensures that materials are efficiently recovered and reused, minimizing waste and conserving resources.
Conclusion: An effective circular supply chain incorporates key components such as sustainable sourcing, efficient production processes, product longevity, reverse logistics, recycling, collaboration, and digital technologies. Engineers play a vital role in designing and optimizing these supply chains to support circular economy principles, ensuring resource efficiency, waste minimization, and sustainability. By integrating circular practices into the supply chain, industries can achieve economic and environmental benefits, enhancing their resilience and competitiveness in a sustainable future.
10. How can lifecycle assessment (LCA) be utilized in green building projects to enhance sustainability, and what are its limitations?
Answer:
Lifecycle Assessment (LCA) is a systematic tool used to evaluate the environmental impacts of a product, process, or system throughout its entire lifecycle—from raw material extraction and manufacturing to use, maintenance, and disposal. In green building projects, LCA helps engineers and designers make informed decisions that enhance sustainability by identifying areas where environmental performance can be improved.
Utilization of LCA in Green Building Projects:
Identifying Environmental Hotspots:
- Action: Conduct LCA to pinpoint stages in the product lifecycle with the highest environmental impacts, such as raw material extraction, manufacturing, transportation, use, and disposal.
- Benefit: Focuses efforts on areas where sustainability improvements can have the most significant impact.
Optimizing Material Selection:
- Action: Use LCA to compare the environmental impacts of different materials and select those with lower embodied energy, reduced emissions, and sustainable sourcing.
- Benefit: Minimizes the environmental footprint of buildings by choosing more sustainable materials.
Enhancing Design for Sustainability:
- Action: Incorporate LCA findings into the design process to create buildings that are easier to reuse, repair, and recycle, thereby extending their lifecycle and reducing waste.
- Benefit: Promotes circularity by ensuring that buildings are designed with their entire lifecycle in mind.
Improving Manufacturing Processes:
- Action: Analyze the environmental impacts of manufacturing processes and identify opportunities to reduce energy consumption, water use, and emissions.
- Benefit: Enhances resource efficiency and lowers the environmental impact of production activities.
Facilitating Resource Recovery:
- Action: Design buildings and systems that facilitate the recovery and reuse of materials at the end of their lifecycle, supported by LCA insights on material flows.
- Benefit: Supports the establishment of closed-loop systems, reducing the need for virgin materials and minimizing waste.
Informing Policy and Strategy:
- Action: Use LCA data to inform corporate sustainability strategies, policy development, and regulatory compliance efforts.
- Benefit: Ensures that sustainability initiatives are data-driven and aligned with environmental goals and regulatory requirements.
Communicating Sustainability Performance:
- Action: Present LCA results to stakeholders, including customers, investors, and regulatory bodies, to demonstrate the environmental performance of buildings.
- Benefit: Enhances transparency, builds trust, and supports marketing efforts focused on sustainability.
Benefits in Industrial Ecology and Circular Economy:
- Holistic View: Provides a comprehensive understanding of environmental impacts across the entire building lifecycle, enabling systemic improvements.
- Data-Driven Decisions: Empowers engineers to make informed decisions based on quantitative data, enhancing the sustainability of buildings and processes.
- Continuous Improvement: Facilitates ongoing evaluation and refinement of buildings and systems to achieve higher levels of sustainability and circularity.
- Integration of Circular Principles: Supports the integration of reuse, recycling, and resource recovery into building design and industrial processes, aligning with circular economy goals.
Limitations of LCA in Green Building Projects:
Data Availability and Quality:
- Limitation: LCA relies on accurate and comprehensive data, which can be difficult to obtain for certain materials or processes.
- Impact: Incomplete or low-quality data can lead to inaccurate assessments and misguided decisions.
Complexity and Time-Consumption:
- Limitation: Conducting a thorough LCA can be complex and time-consuming, requiring specialized knowledge and resources.
- Impact: May delay project timelines and increase costs, particularly for smaller projects or teams with limited expertise.
Scope and Boundary Setting:
- Limitation: Defining the scope and boundaries of the assessment can be challenging, leading to potential omissions or biases.
- Impact: Inconsistent scope definitions can affect the comparability and relevance of LCA results across different projects.
Subjectivity in Impact Assessment:
- Limitation: Assigning weights to different environmental impacts can introduce subjectivity, influencing the overall assessment outcome.
- Impact: Different stakeholders may prioritize impacts differently, leading to varied interpretations of sustainability.
Dynamic Environmental Factors:
- Limitation: LCA typically provides a static analysis and may not account for dynamic changes in environmental conditions or technological advancements.
- Impact: May overlook future improvements or shifts in environmental factors that could affect the building’s sustainability.
Economic and Social Factors:
- Limitation: LCA primarily focuses on environmental impacts and may not fully incorporate economic and social dimensions of sustainability.
- Impact: Provides an incomplete picture of overall sustainability, potentially neglecting important non-environmental factors.
Example:
An LCA conducted for a green office building identifies that using recycled steel significantly reduces the embodied carbon compared to virgin steel. However, the assessment may overlook the social benefits of job creation in the recycling industry or the potential for future technological advancements in steel production that could further enhance sustainability.
Conclusion: Lifecycle Assessment (LCA) is an invaluable tool for engineers seeking to improve the sustainability of green building projects within an industrial ecology framework. By providing a detailed analysis of environmental impacts across the entire building lifecycle, LCA enables engineers to make informed decisions, optimize resource use, and integrate circular economy principles into building design and manufacturing. This leads to the development of more sustainable, efficient, and resilient buildings that contribute to the overall goals of industrial ecology and the circular economy. However, engineers must also be aware of the limitations of LCA, such as data challenges, complexity, and the need to consider economic and social factors, to ensure a comprehensive approach to sustainability.
11. How can engineers assess the social and economic impacts of environmental policies on local communities, and why is this assessment important?
Answer:
Engineers can assess the social and economic impacts of environmental policies on local communities through comprehensive impact assessments, stakeholder engagement, and data analysis. This assessment involves evaluating how policies affect factors such as employment, public health, access to resources, and overall quality of life within communities.
Assessment Methods:
Socio-Economic Impact Assessments (SEIA):
- Description: Systematically evaluates the potential social and economic consequences of environmental policies.
- Application: Analyzing how a new environmental regulation on industrial emissions may affect local employment and public health.
Stakeholder Surveys and Interviews:
- Description: Collects qualitative and quantitative data from community members, businesses, and other stakeholders to understand their perspectives and experiences.
- Application: Conducting surveys to gauge public opinion on the perceived benefits and drawbacks of a renewable energy project.
Economic Modeling:
- Description: Uses statistical and mathematical models to predict the economic impacts of environmental policies, such as changes in GDP, employment rates, and industry growth.
- Application: Modeling the economic effects of implementing a carbon tax on various sectors of the local economy.
Case Studies and Comparative Analysis:
- Description: Examines similar projects or policies in different contexts to identify potential social and economic outcomes.
- Application: Studying the impact of green infrastructure projects in other cities to anticipate outcomes in a new urban development project.
Community Engagement Workshops:
- Description: Facilitates discussions and workshops with community members to identify concerns, expectations, and potential impacts of environmental policies.
- Application: Hosting workshops to discuss the implementation of a new recycling program and its effects on local businesses and residents.
Importance of Assessment:
Informed Decision-Making:
- Benefit: Provides a holistic understanding of how policies affect communities, enabling engineers and policymakers to design more equitable and effective solutions.
- Impact: Ensures that environmental initiatives are socially just and economically viable, fostering broader support and acceptance.
Mitigating Negative Impacts:
- Benefit: Identifies potential adverse effects on vulnerable populations, allowing for the development of mitigation strategies to minimize harm.
- Impact: Protects community well-being and prevents exacerbating existing social and economic inequalities.
Enhancing Policy Acceptance:
- Benefit: Engaging communities and addressing their concerns fosters trust and support for environmental policies, increasing the likelihood of successful implementation.
- Impact: Reduces opposition and facilitates smoother policy adoption and enforcement.
Promoting Sustainable Development:
- Benefit: Ensures that environmental policies contribute to both ecological sustainability and socio-economic well-being, aligning with the principles of sustainable development.
- Impact: Creates a balanced approach to development that benefits both the environment and the community.
Example:
When implementing a new waste management policy, engineers conduct a socio-economic impact assessment to evaluate how the changes will affect local waste collection jobs, household costs, and recycling participation rates. The assessment reveals potential job shifts from manual to automated processes, leading to the development of training programs to support affected workers and educational campaigns to encourage community engagement in recycling efforts.
Conclusion: Assessing the social and economic impacts of environmental policies on local communities is essential for creating balanced and effective environmental management strategies. By utilizing impact assessments, engaging with stakeholders, and employing economic modeling, engineers can understand the broader implications of their projects and policies. This comprehensive approach ensures that environmental initiatives not only protect natural resources but also enhance the well-being and prosperity of the communities they serve.
12. How can data interoperability standards facilitate the integration of diverse environmental datasets, and what are the benefits of achieving seamless data interoperability in environmental engineering projects?
Answer:
Data interoperability standards are frameworks and protocols that enable different data systems and formats to communicate, share, and utilize data seamlessly. In environmental engineering projects, achieving data interoperability is essential for integrating diverse datasets from various sources, enhancing the efficiency and effectiveness of data analysis and decision-making.
Facilitation of Integration:
Standardized Data Formats:
- Function: Establish common data structures and formats (e.g., JSON, XML, CSV) that allow different systems to interpret and use data consistently.
- Benefit: Ensures compatibility between datasets from different sensors, platforms, and organizations, enabling smooth data exchange and aggregation.
Common Metadata Standards:
- Function: Define standardized metadata schemas that describe the context, quality, and structure of datasets.
- Benefit: Enhances data discoverability, usability, and comparability by providing consistent descriptions of data sources and attributes.
APIs and Data Sharing Protocols:
- Function: Develop application programming interfaces (APIs) and protocols (e.g., OGC standards) that facilitate automated data sharing and integration.
- Benefit: Enables real-time data exchange and interoperability between disparate systems, supporting dynamic and collaborative data usage.
Semantic Standards and Ontologies:
- Function: Utilize standardized vocabularies and ontologies to ensure that data meanings are consistently understood across different systems.
- Benefit: Prevents misinterpretation of data by aligning terminologies and concepts, enabling accurate data integration and analysis.
Data Governance Frameworks:
- Function: Implement policies and practices that ensure data quality, security, and compliance with interoperability standards.
- Benefit: Promotes trust and reliability in integrated datasets, ensuring that data can be effectively used for decision-making.
Benefits of Seamless Data Interoperability:
Enhanced Data Accessibility and Sharing:
- Benefit: Facilitates easy access to a wide range of datasets, promoting collaboration among environmental engineers, researchers, and stakeholders.
- Impact: Accelerates the development of comprehensive environmental assessments and solutions by leveraging diverse data sources.
Improved Data Quality and Consistency:
- Benefit: Ensures that integrated datasets are consistent, accurate, and reliable, reducing errors and enhancing the validity of analyses.
- Impact: Leads to more robust and credible environmental engineering outcomes, supporting effective decision-making and policy development.
Increased Efficiency and Reduced Redundancy:
- Benefit: Eliminates the need for manual data conversion and duplication, saving time and resources.
- Impact: Streamlines data workflows, allowing engineers to focus on analysis and application rather than data management.
Facilitated Comprehensive Analysis:
- Benefit: Enables the combination of diverse datasets (e.g., air quality, water quality, land use) for holistic environmental analysis.
- Impact: Provides a more complete understanding of environmental systems, supporting integrated and multifaceted engineering solutions.
Support for Advanced Technologies:
- Benefit: Enhances the effectiveness of machine learning, artificial intelligence, and big data analytics by providing well-integrated and standardized datasets.
- Impact: Unlocks new possibilities for predictive modeling, trend analysis, and innovative environmental engineering applications.
Scalability and Adaptability:
- Benefit: Allows environmental monitoring systems to scale and adapt by easily incorporating new data sources and technologies.
- Impact: Ensures that environmental engineering projects remain flexible and can evolve with emerging data needs and technological advancements.
Example:
An environmental engineering project aiming to assess urban air quality can integrate data from satellite remote sensing, ground-based sensor networks, and traffic monitoring systems using standardized data formats and metadata. This seamless integration allows for comprehensive analysis of pollution sources, dispersion patterns, and the effectiveness of mitigation measures.
Conclusion: Data interoperability standards are crucial for integrating diverse environmental datasets, enabling seamless data sharing, enhancing data quality, and supporting comprehensive analysis in environmental engineering projects. Achieving data interoperability fosters collaboration, increases efficiency, and facilitates the use of advanced technologies, ultimately leading to more effective and informed environmental management and engineering solutions.
Conclusion: The above thought-provoking questions and detailed answers provide a comprehensive exploration of industrial ecology and the circular economy within environmental engineering. Covering fundamental concepts, technological advancements, economic and environmental benefits, interdisciplinary collaboration, and innovative strategies, these inquiries encourage students to critically analyze and engage with the complexities of circular economy practices. By delving into topics such as lifecycle assessment, data interoperability, policy advocacy, and the integration of digital technologies, students are equipped with the knowledge and critical thinking skills necessary to excel in the field of environmental engineering and contribute effectively to sustainable industrial ecosystems. These questions foster a deeper understanding of how circular economy principles can be applied to create resilient, efficient, and sustainable industrial systems, making learning an engaging and dynamic process.