Hawking Radiation and Black Hole Thermodynamics: Bridging Quantum Mechanics and Gravity
The discovery of Hawking radiation revolutionized our understanding of black holes, revealing them to be not entirely black, but capable of emitting radiation due to quantum effects near their boundaries. This insight bridges the realms of physics and astrophysics, merging general relativity with quantum field theory. As a result, black holes—long considered cosmic endpoints—are now seen as dynamic objects with entropy and temperature, giving rise to the vibrant field of black hole thermodynamics.
Understanding Hawking radiation requires exploring the interplay between quantum vacuum fluctuations and event horizons. Near these boundaries, particle-antiparticle pairs are spontaneously generated. One particle may fall into the black hole while the other escapes, appearing as thermal radiation to an outside observer. This thermodynamic behavior of black holes not only relates to entropy but also supports the idea that black holes gradually lose mass, suggesting a possible endpoint where they fully evaporate.
This thermodynamic evolution invites parallels with classical systems governed by classical mechanics and deeper insights into analytical mechanics. However, the paradoxes that arise—such as the information loss problem—highlight fundamental conflicts between relativity and quantum theory, urging the development of a unified theory of quantum gravity.
The connection between black holes and dark matter is particularly intriguing when examined through thermodynamic lenses. Some hypotheses suggest that Hawking radiation may be influenced by the presence of dark matter, potentially altering black hole lifespans or contributing to our indirect detection of this elusive substance. The interaction between black holes and dark matter is still largely speculative but remains a hot topic in theoretical models.
In the grand narrative of cosmology, Hawking radiation ties into early-universe scenarios such as the Big Bang Theory and the emergence of microscopic black holes. The cosmic microwave background and the study of dark energy continue to inform our understanding of how thermodynamic principles shape the structure and evolution of the universe.
The intersection of black hole radiation and entropy also prompts speculation about the multiverse and conditions of nuclear fusion within extreme environments. The thermodynamic laws that govern black holes are linked to the energy balance in stellar physics, echoing through star life cycles and the final states of matter in neutron stars and white dwarfs.
Observational astrophysics is gradually catching up to these theories. Spectral patterns from stellar atmospheres, data from variable stars, and principles drawn from celestial mechanics and continuum mechanics are employed to refine models and detect signatures of evaporation or entropy changes. These efforts further link thermodynamic phenomena to the life and fate of celestial bodies.
Ultimately, Hawking radiation and black hole thermodynamics offer a compelling lens through which to view the universe—not just as a collection of objects and events, but as a dynamic, evolving system governed by principles that unify the smallest quantum fluctuations with the grandest cosmic structures.
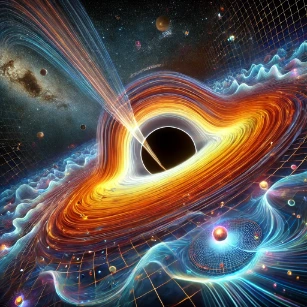
Table of Contents
Hawking Radiation: Quantum Emission from Black Holes
Origin of the Concept
Stephen Hawking, in 1974, combined principles from quantum field theory and general relativity to show that black holes can emit radiation. This phenomenon, now known as Hawking radiation, arises due to quantum fluctuations near the event horizon.Quantum Vacuum Fluctuations
- Quantum Uncertainty: According to the Heisenberg Uncertainty Principle, the vacuum of space is not truly empty but teems with fleeting particle-antiparticle pairs that spontaneously form and annihilate.
- Pair Production near the Event Horizon:
Near a black hole’s event horizon, these virtual pairs can be separated by the immense gravitational field.
- One particle falls into the black hole, while the other escapes into space.
- The escaping particle becomes real and is detected as Hawking radiation.
Negative Energy and Black Hole Mass Loss
- The particle that falls into the black hole carries negative energy (from the perspective of an external observer), effectively reducing the black hole’s mass.
- Over astronomical timescales, this process leads to black hole evaporation.
- Smaller black holes emit more radiation and lose mass faster due to the inverse relationship between mass and temperature.
Mathematical Expression
The temperature of Hawking radiation, also called the Hawking Temperature (Tₕ), is inversely proportional to the black hole’s mass: Where:- ℏ is the reduced Planck constant.
- c is the speed of light
- G is the gravitational constant.
- M is the mass of the black hole.
- KB is Boltzmann’s constant
End Stage of Black Hole Evaporation
- As the black hole loses mass, its temperature increases, accelerating the evaporation process.
- Theoretical models predict that a black hole could end in a violent burst of gamma rays during its final moments.
- This terminal explosion could potentially be detected, offering evidence for Hawking radiation.
Black Hole Thermodynamics: Uniting Gravity and Heat
Black hole thermodynamics extends the laws of classical thermodynamics to black holes, providing a profound link between entropy, energy, and spacetime geometry. Before Hawking’s discovery, black holes were thought to violate thermodynamic principles, as nothing could escape, seemingly preventing heat transfer or entropy exchange.The Four Laws of Black Hole Thermodynamics
Analogous to the classical laws of thermodynamics, black hole thermodynamics introduces corresponding laws to describe the behavior of black holes:First Law (Energy Conservation)
- Analogous to the First Law of Thermodynamics (ΔU = Q – W), where energy is conserved.
- Changes in the black hole’s mass (M) relate to changes in its area (A), angular momentum (J), and electric charge (Q).
- κ: Surface gravity.
- Ω: Angular velocity.
- Φ: Electric potential.
Second Law (Entropy Increase)
- The surface area of a black hole’s event horizon never decreases.
- This mirrors the classical Second Law of Thermodynamics, where the total entropy of an isolated system never decreases.
- Jacob Bekenstein first proposed that a black hole’s entropy is proportional to the area of its event horizon:
- S is entropy,
- A is the event horizon area.
Third Law (Absolute Zero)
- It is impossible to reduce a black hole’s surface gravity to zero through any finite process.
- This mirrors the classical statement that absolute zero temperature is unattainable.
Zeroth Law (Thermal Equilibrium)
- A black hole in equilibrium has a constant surface gravity across its event horizon, akin to constant temperature in thermodynamic systems.
Implications of Hawking Radiation and Thermodynamics
Black Hole Evaporation and Lifespan
- Massive black holes emit negligible Hawking radiation due to their large mass and low temperature.
- Micro black holes (hypothetical or primordial) could evaporate more rapidly and might have already vanished.
- Hawking radiation implies that no black hole is eternal. Over time, all black holes could eventually evaporate, leaving behind only radiation.
The Black Hole Information Paradox
- Hawking’s theory suggests that information about matter falling into a black hole is destroyed when the black hole evaporates.
- This conflicts with quantum mechanics, which dictates that information cannot be lost, leading to the black hole information paradox.
- Proposed Solutions:
- Holographic Principle: Suggests that information is encoded on the event horizon.
- Firewall Hypothesis: Proposes a high-energy boundary at the event horizon.
- Information leakage via Hawking radiation, though not yet fully understood.
Link Between Gravity and Quantum Mechanics
- Hawking radiation bridges general relativity (describing gravity) and quantum field theory, highlighting the need for a unified theory of quantum gravity.
- Black holes offer natural laboratories to test this connection.
Thermodynamic Arrow of Time
- The entropy associated with black holes supports the thermodynamic arrow of time, reinforcing the irreversible nature of processes in the universe.
Observational Challenges and Prospects
Detection of Hawking Radiation
- For stellar-mass and supermassive black holes, Hawking radiation is undetectable due to its incredibly low temperature.
- Primordial black holes, if they exist, could emit detectable bursts of radiation during their final evaporation stages.
- Future detectors might observe gamma-ray bursts or other high-energy signals as potential evidence.
Experimental Analogues
- Laboratory analogs using Bose-Einstein condensates and optical fibers attempt to simulate conditions similar to black hole event horizons, offering indirect ways to study Hawking radiation.
Why Study Hawking Radiation and Black Hole Thermodynamics
Quantum Mechanics in Curved Spacetime
Hawking radiation describes how black holes emit particles due to quantum effects near the event horizon. Students explore how quantum theory interacts with gravitational fields. This concept merges general relativity with quantum mechanics. It illustrates how the universe behaves at its most fundamental level.
Thermodynamic Properties of Black Holes
Students learn that black holes have temperature, entropy, and other thermodynamic attributes. This challenges previous notions of black holes as purely absorptive objects. It reveals deep connections between gravity, entropy, and energy. It opens new directions in theoretical physics.
Information Loss and Quantum Paradoxes
Hawking radiation raises questions about whether information is destroyed when black holes evaporate. Students examine the information paradox and proposed solutions. These discussions lie at the heart of efforts to unify physics. They foster critical thinking about the nature of reality.
Research Applications and Theoretical Advances
Black hole thermodynamics has inspired developments in quantum field theory, string theory, and holography. Students study how these frameworks explain Hawking radiation. This research advances our understanding of fundamental forces and particles. It trains students in frontier theoretical work.
Contribution to Unifying Physics
Hawking radiation represents a key step toward reconciling gravity and quantum theory. Students working in this area engage with some of the most profound challenges in science. It is a gateway to careers in theoretical physics and cosmology. It offers the chance to shape future scientific understanding.
Hawking Radiation and Thermodynamics: Conclusion
Hawking radiation and black hole thermodynamics have revolutionized our understanding of black holes, transforming them from purely gravitational phenomena into thermodynamic and quantum systems. Hawking’s theory revealed that black holes emit radiation, slowly evaporate, and possess temperature and entropy, directly linking quantum mechanics, thermodynamics, and general relativity. These discoveries pose profound questions about the nature of information, entropy, and the ultimate fate of black holes and the universe. While direct detection of Hawking radiation remains elusive, ongoing research continues to explore the deepest connections between the fundamental forces of nature, pushing us closer to a unified theory of physics.Hawking Radiation and Thermodynamics: Review Questions and Answers:
What is Hawking Radiation?
Answer: Hawking Radiation is a theoretical prediction that black holes emit thermal radiation due to quantum effects near their event horizons. This phenomenon arises from virtual particle pairs forming near the boundary, with one particle escaping while the other falls in, leading to a gradual loss of mass.What is meant by black hole thermodynamics?
Answer: Black hole thermodynamics is the study of the thermodynamic properties of black holes, relating quantities such as temperature, entropy, and energy. It draws parallels between the laws of thermodynamics and the behavior of black holes, suggesting that they have measurable thermal characteristics despite their extreme gravitational pull.How does Hawking Radiation arise from quantum effects near the event horizon?
Answer: Near a black hole’s event horizon, quantum fluctuations allow for the spontaneous creation of particle-antiparticle pairs. When one particle escapes and the other is captured by the black hole, the escaping particle appears as radiation. This process results in a net energy loss from the black hole, manifesting as Hawking Radiation.Why is the concept of black hole temperature significant?
Answer: The black hole temperature, as predicted by Hawking Radiation, is significant because it quantifies the thermal emission from a black hole. This temperature, which is inversely proportional to the black hole’s mass, links gravitational phenomena with quantum theory, suggesting that black holes behave like ordinary thermodynamic objects.How is entropy defined in the context of black hole thermodynamics?
Answer: In black hole thermodynamics, entropy is proportional to the area of the event horizon rather than the volume. This relationship, known as the Bekenstein-Hawking entropy, implies that the information content of a black hole is encoded on its surface, challenging conventional ideas about information storage in physical systems.What is the black hole information paradox?
Answer: The information paradox arises from the question of whether information that falls into a black hole is permanently lost when the black hole evaporates through Hawking Radiation. This challenges the principles of quantum mechanics, which dictate that information must be conserved, leading to ongoing debates about the reconciliation of quantum theory and general relativity.How do quantum fluctuations contribute to the emission of Hawking Radiation?
Answer: Quantum fluctuations near the event horizon lead to the transient appearance of virtual particle pairs. When these pairs are produced, the intense gravitational field can separate them, allowing one particle to escape as Hawking Radiation while the other is absorbed by the black hole, effectively converting vacuum energy into observable radiation.What role does the uncertainty principle play in black hole thermodynamics?
Answer: The uncertainty principle, which limits the precision of simultaneous measurements of certain pairs of observables, underpins the formation of virtual particles near the event horizon. This principle allows for temporary violations of energy conservation that enable the creation of particle pairs, thereby driving the process of Hawking Radiation and influencing the thermal properties of black holes.How does Hawking Radiation lead to the gradual evaporation of black holes?
Answer: As Hawking Radiation carries energy away from the black hole, it results in a loss of mass over time. This mass loss causes the black hole to shrink and, theoretically, to eventually evaporate completely if no additional mass is accreted, a process that has significant implications for the long-term evolution of black holes.What challenges exist in detecting Hawking Radiation?
Answer: Detecting Hawking Radiation is challenging due to its extremely weak signal, especially from large black holes whose temperatures are very low. Additionally, the cosmic background noise and the need for highly sensitive instruments make it difficult to distinguish this radiation from other astrophysical sources, necessitating advanced observational technologies and methods.
Hawking Radiation and Thermodynamics: Thought-Provoking Questions and Answers
How might breakthroughs in gravitational wave astronomy alter our understanding of event horizons and Hawking Radiation?
Answer: Breakthroughs in gravitational wave astronomy could provide new insights into the dynamics of black hole mergers and the behavior of event horizons. Detecting subtle gravitational wave signals associated with quantum effects near black holes may offer indirect evidence of Hawking Radiation, refining our theoretical models and enhancing our understanding of the interplay between gravity and quantum mechanics.What new physics could emerge from a deeper understanding of the information paradox?
Answer: A deeper understanding of the information paradox could lead to revolutionary theories in quantum gravity, potentially resolving inconsistencies between quantum mechanics and general relativity. It might reveal novel mechanisms for information preservation, such as holographic principles or entanglement structures, and prompt the development of a unified framework that accommodates both quantum and gravitational phenomena.How might future observational technologies detect the minute signals of Hawking Radiation?
Answer: Future observational technologies, such as ultra-sensitive space-based detectors or advanced interferometers, could potentially detect the faint thermal emissions predicted by Hawking Radiation. Improvements in detector sensitivity, noise reduction techniques, and long-duration observation campaigns might make it possible to observe these subtle signals, providing empirical evidence for quantum effects near black holes.What are the implications of black hole thermodynamics for the ultimate fate of black holes?
Answer: Black hole thermodynamics suggests that black holes are not eternal but will eventually evaporate through the emission of Hawking Radiation. This implies that over extremely long timescales, black holes could lose all their mass and vanish, leading to profound questions about the end state of these objects and the fate of the information they contain.How could modifications to the standard model of particle physics impact our understanding of Hawking Radiation?
Answer: If modifications to the standard model introduce new particles or interactions, they could affect the spectrum and intensity of Hawking Radiation. Such changes might alter the predicted temperature and evaporation rate of black holes, necessitating revisions to our current theories and potentially offering clues about the underlying quantum structure of spacetime.In what ways does the concept of entropy in black hole thermodynamics challenge conventional notions of information storage?
Answer: The concept that black hole entropy is proportional to the surface area rather than the volume challenges traditional ideas of information storage, suggesting that all the information about the interior of a black hole is encoded on its boundary. This holographic principle has far-reaching implications for our understanding of quantum gravity and the nature of spacetime, prompting reevaluation of how information is fundamentally organized in the universe.What role might quantum entanglement play in resolving the black hole information paradox?
Answer: Quantum entanglement could play a critical role in ensuring that information is not lost when matter falls into a black hole. If the emitted Hawking Radiation is entangled with the information contained within the black hole, it might be possible to reconstruct the lost data, thereby preserving the principles of quantum mechanics and offering a solution to the information paradox.How do virtual particles near the event horizon challenge our classical understanding of vacuum and emptiness?
Answer: The concept of virtual particles emerging from quantum fluctuations near the event horizon challenges the classical notion of a vacuum as an empty space. Instead, it reveals a dynamic and energetic environment where particle-antiparticle pairs constantly form and annihilate, suggesting that even seemingly empty space is filled with transient activity that has measurable consequences, such as Hawking Radiation.What potential does the study of black hole thermodynamics hold for advancing our search for a theory of quantum gravity?
Answer: Studying black hole thermodynamics provides a unique intersection of quantum mechanics and general relativity, offering empirical challenges that any theory of quantum gravity must address. Insights from black hole entropy, temperature, and radiation processes could guide the development of new theoretical models that reconcile the two frameworks, ultimately leading to a deeper, unified understanding of the fundamental forces.How might our understanding of black hole evaporation influence cosmological models of the universe’s long-term evolution?
Answer: Understanding black hole evaporation through Hawking Radiation could have significant implications for cosmology, particularly in predicting the long-term behavior of the universe. If black holes evaporate completely, this process might affect the distribution of energy and matter over cosmic timescales, influencing theories about the ultimate fate of the universe and the evolution of cosmic structures.What experimental setups could be designed to simulate Hawking Radiation in a laboratory environment?
Answer: Experimental setups such as analog gravity systems, where fluid dynamics or optical systems mimic the behavior of spacetime near a black hole, could be used to simulate Hawking Radiation. By replicating the conditions that lead to particle pair creation and energy emission, researchers may be able to observe analogous effects in the lab, providing valuable insights into the quantum processes at play near event horizons.How can public outreach and science communication be enhanced to explain complex concepts like Hawking Radiation and black hole thermodynamics to a broader audience?
Answer: Enhancing public outreach can be achieved through interactive visualizations, engaging multimedia content, and accessible analogies that relate complex astrophysical concepts to everyday experiences. By using educational platforms, public lectures, and virtual reality simulations, scientists can demystify these topics, inspiring curiosity and fostering a deeper public appreciation for the profound questions underlying the nature of our universe.