Evolutionary Biology
Evolutionary biology is the foundational discipline that explores how life has diversified and adapted through time. It builds on principles from science and draws extensively from biology to understand the origin and transformation of species. By tracing genetic, molecular, and ecological changes across generations, evolutionary biology offers a comprehensive view of life’s dynamic history. Insights from ecology help contextualize how environmental pressures influence evolutionary outcomes, while studies in cell biology provide a microscopic view of the structures and processes that undergo evolutionary modifications.
Evolutionary patterns become clearer through examining the roles of cell communication, cell cycles, and cell development, all of which contribute to organismal variation and heredity. Internal regulatory processes explored in cell physiology and the anatomical framework described in cell structure also reveal important evolutionary adaptations.
A core component of evolutionary biology is genetics, which investigates how traits are inherited and modified. This includes classical studies in Mendelian genetics and modern fields such as genomics. The mechanisms behind inheritance are unraveled through molecular genetics, where the roles of DNA and RNA, DNA technology, and gene expression are closely examined.
Variation within populations, driven by genetic mutation, sets the stage for natural selection. Understanding the molecular basis of inheritance clarifies how such variations are passed on, while molecular evolution provides a timeline for these genetic changes. The use of molecular techniques in research continues to enhance our understanding of evolutionary processes by enabling precise comparisons across species and time.
The production of proteins, critical to phenotypic traits, is detailed in protein synthesis studies. How these proteins affect traits within populations is further explored in population genetics and modeled through quantitative genetics. Real-world implications of evolutionary insights are evident in applications of genetics in medicines, which use evolutionary principles to predict disease resistance and inform personalized treatment plans.
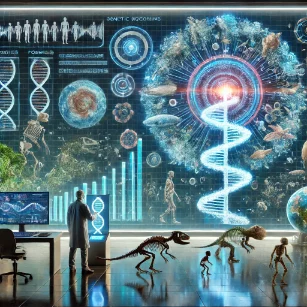
Table of Contents
Key Objectives of Evolutionary Biology
Understanding the Mechanisms of Evolutionary Change: Investigating the processes of mutation, natural selection, genetic drift, and gene flow that drive evolution at the genetic and phenotypic levels.
Tracing the History of Life: Reconstructing the evolutionary relationships among species and identifying common ancestors through the study of fossils, comparative anatomy, and molecular data.
Exploring Adaptation: Examining how organisms evolve traits that enhance survival and reproduction in their specific environments.
Explaining Biodiversity: Understanding how speciation and extinction shape the diversity of life over time.
Connecting Microevolution and Macroevolution: Studying how small-scale genetic changes within populations (microevolution) contribute to large-scale evolutionary patterns, such as the emergence of new taxa (macroevolution).
Foundations of Evolutionary Biology
Darwin’s Theory of Evolution by Natural Selection: In 1859, Charles Darwin’s On the Origin of Species laid the foundation for understanding evolution. Darwin proposed that:
- Species evolve through gradual change over time.
- Natural selection acts on heritable variations, favoring traits that improve survival and reproduction.
Mendelian Genetics and the Modern Synthesis: In the early 20th century, the integration of Darwinian evolution with Mendelian genetics formed the Modern Synthesis. This framework explained how genetic inheritance and variation underlie evolutionary change.
Molecular Evolution: The discovery of DNA as the molecule of inheritance in the mid-20th century allowed evolutionary biologists to study genetic changes at the molecular level, providing deeper insights into evolutionary mechanisms.
Neutral Theory and Genetic Drift: Motoo Kimura’s neutral theory of molecular evolution (1968) highlighted the role of random genetic changes (neutral mutations) and genetic drift in shaping genetic diversity.
Mechanisms of Evolution
Natural Selection: The process by which certain traits become more common in a population because they confer advantages in survival or reproduction.
Mutation: Random changes in DNA sequences introduce new genetic variation, providing the raw material for evolution.
Genetic Drift: Random fluctuations in allele frequencies, especially in small populations, can lead to evolutionary change.
Gene Flow: The movement of genes between populations through migration promotes genetic diversity and prevents populations from becoming genetically isolated.
Speciation: The formation of new species occurs wh
Major Themes in Evolutionary Biology
Adaptation and Natural Selection:
- Adaptive traits improve an organism’s fitness in its environment.
- Examples include the beak shapes of Darwin’s finches and the camouflage of peppered moths during the Industrial Revolution.
Evolutionary Developmental Biology (Evo-Devo):
- Examines how changes in developmental genes and pathways drive evolutionary changes in body plans and structures.
- For example, changes in Hox genes are linked to the diversification of animal forms.
Molecular Evolution:
- Studies changes at the DNA, RNA, and protein levels to understand evolutionary relationships and functional adaptations.
- Examples include the evolution of antibiotic resistance and the origins of genetic novelty.
Phylogenetics and Systematics:
- Reconstructing the evolutionary tree of life to map relationships among species.
- Molecular phylogenetics uses DNA and protein sequences to infer evolutionary histories.
Coevolution:
- Examines reciprocal evolutionary influences between interacting species, such as predator-prey dynamics, host-parasite relationships, and mutualism (e.g., flowers and pollinators).
Human Evolution:
- Investigates the origins of modern humans, including the evolution of bipedalism, brain size, and culture.
- Explores genetic evidence from ancient DNA and fossils.
Extinction and Mass Extinctions:
- Studies the causes and consequences of species extinction, including past mass extinction events like the one that wiped out the dinosaurs.
Broader Applications of Evolutionary Biology
-
Medicine:
- Understanding the evolution of pathogens informs strategies to combat antibiotic resistance and emerging diseases.
- Insights into human genetics and evolution aid in personalized medicine and understanding genetic disorders.
-
Agriculture:
- Evolutionary principles guide the development of pest-resistant crops and sustainable farming practices.
- Artificial selection in crop and livestock breeding mimics natural selection.
-
Conservation Biology:
- Evolutionary biology helps prioritize species for conservation based on genetic diversity and evolutionary potential.
- Understanding evolutionary processes aids in restoring ecosystems and managing invasive species.
-
Biotechnology:
- Directed evolution techniques optimize enzymes and other biomolecules for industrial and medical applications.
-
Climate Change and Adaptation:
- Studying evolutionary responses to changing environments helps predict the adaptability of species to climate change.
Why Study Evolutionary Biology
Explaining the Diversity of Life
Evolutionary biology explores how species have changed over time through natural selection and genetic variation. It provides the framework for understanding biological diversity. This knowledge connects all branches of biology, from genetics to ecology. It helps students appreciate the unity and complexity of life.
Foundations of Modern Biology
The theory of evolution underpins concepts in genetics, development, and behavior. It explains how traits are inherited and how populations adapt to environments. Students learn how evolutionary processes shape life on Earth. This makes it essential for any biological or biomedical education.
Insights into Human Origins
Evolutionary biology provides evidence for the origin and development of Homo sapiens. It helps students understand the biological and cultural factors that make us human. This knowledge is relevant in anthropology, medicine, and public health. It promotes a deeper understanding of our place in the natural world.
Applications in Medicine and Biotechnology
Understanding evolution is crucial for addressing antibiotic resistance, vaccine development, and emerging diseases. It informs strategies to combat rapidly evolving pathogens. This makes evolutionary biology important for careers in medicine and global health. It also supports advancements in genetic engineering and personalized medicine.
Research and Analytical Thinking
Evolutionary biology develops skills in critical thinking, hypothesis testing, and data analysis. Students engage with fossil records, molecular phylogenies, and population models. These skills are transferable to many scientific and analytical fields. They empower students to explore the patterns and processes of life scientifically.
The Future of Evolutionary Biology
Evolutionary biology is a vibrant and expanding field, continuously reshaped by new discoveries and technologies. Advancements in genomics, bioinformatics, and computational modeling have opened up new avenues for studying evolution on unprecedented scales. For example, ancient DNA research has transformed our understanding of human prehistory, while genome-wide association studies reveal the genetic basis of adaptation in diverse organisms.
As we face global challenges like biodiversity loss and climate change, evolutionary biology offers critical insights into how species adapt and ecosystems respond to environmental pressures. It remains central to our understanding of life’s past, present, and future, offering a unifying framework for all of biology.
Evolutionary Biology: Review Questions and Answers
Question 1:
What is the theory of natural selection, and how does it drive evolutionary change in populations?
Answer:
Theory of Natural Selection Defined:
Natural selection is a fundamental mechanism of evolution proposed by Charles Darwin. It explains how populations of organisms change over generations through the differential survival and reproduction of individuals with advantageous traits.
Key Components of Natural Selection:
Variation: Individuals within a population exhibit variations in their traits (e.g., size, coloration, speed).
Inheritance: Some of these traits are heritable and can be passed from parents to offspring.
Differential Survival and Reproduction: Individuals with traits better suited to their environment are more likely to survive and reproduce, passing those advantageous traits to the next generation.
Adaptation: Over time, beneficial traits become more common in the population, leading to adaptation to the environment.
How Natural Selection Drives Evolutionary Change:
Environmental Pressure: Changes in the environment create selective pressures that favor certain traits over others.
Fitness Advantage: Traits that enhance an organism’s fitness (ability to survive and reproduce) increase in frequency.
Population Shift: As advantageous traits become more prevalent, the overall genetic makeup of the population shifts, resulting in evolutionary change.
Speciation: Prolonged natural selection can lead to the emergence of new species as populations diverge genetically.
Example:
- Peppered Moth (Biston betularia): In England, industrial pollution darkened tree trunks, favoring darker-colored moths that were less visible to predators. As a result, the frequency of dark moths increased in polluted areas, illustrating natural selection in action.
Conclusion:
Natural selection is a cornerstone of evolutionary biology, driving the adaptation and diversification of life by favoring traits that enhance survival and reproductive success in specific environments.
Question 2:
Explain the concept of genetic drift and its role in evolutionary processes.
Answer:
Genetic Drift Defined:
Genetic drift is a mechanism of evolution that involves random changes in allele frequencies within a population. Unlike natural selection, which is driven by environmental pressures and differential fitness, genetic drift occurs by chance and can significantly impact small populations.
Key Aspects of Genetic Drift:
Random Sampling: Genetic drift results from random sampling of alleles during reproduction, where some alleles may be passed on more frequently purely by chance.
Population Size: The effects of genetic drift are more pronounced in small populations because random events can lead to significant changes in allele frequencies.
Allele Fixation and Loss: Over time, genetic drift can lead to the fixation (allele frequency reaches 100%) or complete loss (allele frequency reaches 0%) of alleles in a population.
Types of Genetic Drift:
Bottleneck Effect:
- Definition: Occurs when a population undergoes a drastic reduction in size due to events like natural disasters, leading to a loss of genetic diversity.
- Impact: The surviving population may have different allele frequencies than the original population, potentially reducing genetic variation.
Founder Effect:
- Definition: Happens when a new population is established by a small number of individuals from a larger population.
- Impact: The new population may have allele frequencies that differ from the original population, leading to reduced genetic diversity and potential for unique evolutionary paths.
Role of Genetic Drift in Evolutionary Processes:
Neutral Evolution: Genetic drift can drive evolutionary changes in alleles that do not confer any selective advantage or disadvantage (neutral alleles).
Genetic Diversity: Drift can reduce genetic diversity within populations, making them more susceptible to inbreeding and reducing the potential for future adaptive evolution.
Speciation: In isolated small populations, genetic drift can lead to significant genetic divergence, contributing to the formation of new species.
Example:
- Northern Elephant Seals: Once reduced to as few as 20 individuals due to hunting, their current populations show extremely low genetic diversity, a consequence of the bottleneck effect caused by genetic drift.
Conclusion:
Genetic drift is a crucial evolutionary mechanism, especially in small populations, influencing allele frequencies and contributing to genetic diversity and speciation. It operates independently of natural selection, highlighting the role of chance in the evolutionary trajectory of populations.
Question 3:
Describe the process of speciation and the different modes by which new species can arise.
Answer:
Speciation Defined:
Speciation is the evolutionary process through which new distinct species arise from existing ones. It involves the divergence of populations to the point where they can no longer interbreed and produce fertile offspring.
Modes of Speciation:
Allopatric Speciation:
- Definition: Occurs when populations are geographically separated, leading to reproductive isolation.
- Process:
- Geographic Isolation: Physical barriers (mountains, rivers, distance) split a population into separate groups.
- Genetic Divergence: Isolated populations accumulate genetic differences through mutation, genetic drift, and natural selection.
- Reproductive Isolation: Over time, genetic and behavioral differences prevent interbreeding even if populations come into contact again.
- Example: The formation of the Grand Canyon led to the divergence of North American and Arizona populations of the Kaibab squirrel.
Sympatric Speciation:
- Definition: Occurs within a single geographic area without physical barriers, often driven by ecological, behavioral, or genetic factors.
- Process:
- Ecological Niches: Different groups exploit different resources or habitats within the same area.
- Behavioral Isolation: Changes in mating preferences or behaviors lead to reproductive isolation.
- Polyploidy (in plants): Duplication of the chromosome number creates reproductive barriers.
- Example: Apple maggot flies (Rhagoletis pomonella) shifted from hawthorn to apple trees, leading to reproductive isolation and speciation.
Peripatric Speciation:
- Definition: A form of allopatric speciation where a small peripheral population becomes isolated from the main population.
- Process:
- Founder Effect: A small group establishes a new population, leading to rapid genetic changes due to drift.
- Genetic Divergence: The isolated population evolves independently, accumulating unique genetic traits.
- Reproductive Isolation: Differences become significant enough to prevent interbreeding with the original population.
- Example: The Galápagos finches evolved into multiple species from a small ancestral population.
Parapatric Speciation:
- Definition: Occurs when populations are adjacent to each other with a narrow zone of overlap, leading to speciation without complete geographic isolation.
- Process:
- Environmental Gradients: Differences in environmental conditions across a continuous habitat create distinct selective pressures.
- Limited Gene Flow: Restricted interbreeding occurs at the boundary, allowing populations to diverge genetically.
- Reproductive Isolation: Over time, genetic differences lead to reproductive barriers.
- Example: Grasshopper species along different elevations in the same mountain range develop distinct traits adapted to their specific environments.
Mechanisms Facilitating Speciation:
Genetic Mutations: Introduce new genetic variations.
Natural Selection: Favors traits that enhance survival and reproduction in specific environments.
Genetic Drift: Random changes in allele frequencies, especially in small populations.
Reproductive Isolation: Prevents gene flow between diverging populations, allowing independent evolution.
Implications of Speciation:
Biodiversity: Speciation increases the number of species, contributing to the richness and complexity of ecosystems.
Adaptation: New species can occupy different ecological niches, enhancing ecosystem resilience and functionality.
Evolutionary Innovation: Speciation drives the emergence of novel traits and adaptations, fueling evolutionary progress.
Conclusion:
Speciation is a dynamic and multifaceted process essential for the diversification of life on Earth. Understanding the different modes of speciation—allopatric, sympatric, peripatric, and parapatric—provides insights into how new species emerge and adapt, shaping the evolutionary landscape of biodiversity.
Question 4:
What is genetic drift, and how does it differ from natural selection in shaping genetic variation within populations?
Answer:
Genetic Drift Defined:
Genetic drift is a mechanism of evolution that involves random changes in allele frequencies within a population. Unlike natural selection, which is driven by environmental pressures and differential fitness, genetic drift occurs by chance and can significantly impact small populations.
Key Characteristics of Genetic Drift:
Random Sampling: Genetic drift results from random sampling of alleles during reproduction, where some alleles may be passed on more frequently purely by chance.
Population Size: The effects of genetic drift are more pronounced in small populations because random events can lead to significant changes in allele frequencies.
Allele Fixation and Loss: Over time, genetic drift can lead to the fixation (allele frequency reaches 100%) or complete loss (allele frequency reaches 0%) of alleles in a population.
Differences Between Genetic Drift and Natural Selection:
Mechanism:
- Genetic Drift: Driven by random chance without regard to the alleles’ effects on fitness.
- Natural Selection: Driven by differential survival and reproduction based on allele advantages or disadvantages in a given environment.
Directionality:
- Genetic Drift: Allele frequency changes are random and can increase or decrease alleles regardless of their impact on fitness.
- Natural Selection: Changes in allele frequencies are non-random and typically favor alleles that enhance fitness.
Impact on Genetic Variation:
- Genetic Drift: Can reduce genetic variation by randomly eliminating alleles, especially harmful or neutral ones.
- Natural Selection: Can increase genetic variation by favoring beneficial alleles and removing deleterious ones, but can also reduce variation if selection is strong.
Population Size Sensitivity:
- Genetic Drift: More significant in small populations where random events can drastically alter allele frequencies.
- Natural Selection: Operates in populations of all sizes, but its effects are more easily observed in larger populations where selection pressures consistently favor certain alleles.
Examples Illustrating the Differences:
Genetic Drift Example: In a small population of beetles, a random storm may kill individuals regardless of their color, leading to a random change in the frequency of dark and light-colored beetles.
Natural Selection Example: In a large population of beetles, dark-colored individuals may be better camouflaged against predators in a polluted environment, leading to an increase in dark-colored beetles over generations.
Interactions Between Genetic Drift and Natural Selection:
In small populations, genetic drift can overpower natural selection, leading to the fixation of neutral or even deleterious alleles.
In large populations, natural selection generally has a more substantial influence, effectively promoting advantageous alleles and eliminating harmful ones.
Conclusion:
Genetic drift and natural selection are both fundamental evolutionary mechanisms shaping genetic variation within populations. While genetic drift operates through random chance, particularly impacting small populations, natural selection acts non-randomly based on allele fitness. Understanding both processes is essential for comprehending the complexities of evolutionary change and the maintenance of genetic diversity in populations.
Question 5:
How does gene flow contribute to genetic diversity, and what are its potential impacts on speciation?
Answer:
Gene Flow Defined:
Gene flow, also known as gene migration, is the transfer of genetic material (alleles) between separate populations of the same species. It occurs when individuals from one population migrate to another, interbreed, and introduce new alleles into the gene pool.
Contribution of Gene Flow to Genetic Diversity:
- Introduction of New Alleles:
- Allelic Variation: Gene flow introduces alleles that may be absent or rare in the recipient population, increasing genetic diversity.
- Reducing Genetic Differences:
- Homogenization: By mixing gene pools, gene flow reduces genetic differentiation between populations, promoting genetic similarity.
- Enhancing Adaptive Potential:
- Increased Variation: Higher genetic diversity provides a broader range of traits that may enhance a population’s ability to adapt to environmental changes.
Mechanisms Facilitating Gene Flow:
Migration: Movement of individuals between populations through dispersal or migration.
Hybridization: Interbreeding between different populations, often following secondary contact after geographic separation.
Impacts of Gene Flow on Speciation:
Counteracting Speciation:
- Preventing Divergence: Gene flow can inhibit speciation by maintaining genetic similarity between populations, preventing the accumulation of genetic differences necessary for reproductive isolation.
- Example: Continuous migration between two populations can keep allele frequencies similar, reducing the likelihood of speciation.
Facilitating Speciation:
- Hybrid Vigor: In some cases, gene flow can introduce beneficial alleles that enhance fitness, promoting divergence if certain traits are selected.
- Adaptive Introgression: Gene flow can transfer advantageous genes from one population to another, aiding adaptation and potentially contributing to speciation if these genes lead to reproductive isolation.
- Example: Introgression of genes for pesticide resistance from one insect population to another can lead to ecological specialization and eventual speciation.
Maintaining Species Boundaries:
- Reinforcement: In regions where gene flow occurs between diverging populations, natural selection may strengthen reproductive barriers to maintain species integrity.
- Example: If hybrids have reduced fitness, selection may favor traits that prevent interbreeding, reinforcing speciation.
Creating Genetic Clines:
- Gradual Changes: Gene flow can lead to gradual changes in allele frequencies across geographic gradients, resulting in clinal variation rather than discrete speciation.
- Example: Gradual changes in allele frequencies of a trait across a continent due to continuous gene flow can create a cline without leading to distinct species.
Factors Influencing the Role of Gene Flow in Speciation:
Population Size: Smaller populations are more susceptible to genetic drift, potentially overriding the homogenizing effects of gene flow.
Reproductive Barriers: Prezygotic and postzygotic barriers can limit gene flow, facilitating speciation by reducing interbreeding.
Environmental Heterogeneity: Diverse environments can create different selective pressures, allowing gene flow to introduce beneficial alleles that promote local adaptation and divergence.
Frequency of Migration: High rates of migration increase gene flow, making speciation less likely, whereas low rates allow populations to diverge.
Examples Illustrating Gene Flow’s Impact:
African Cichlid Fishes: Gene flow between geographically separated lakes has been shown to introduce new genetic variations, facilitating rapid speciation and adaptive radiation.
Humans: Historical gene flow between different human populations has increased genetic diversity, promoting resilience against diseases and environmental changes.
Conclusion:
Gene flow plays a critical role in shaping genetic diversity within and between populations. While it can counteract the processes leading to speciation by maintaining genetic similarity, it can also facilitate speciation under certain conditions by introducing beneficial alleles and promoting adaptive divergence. Understanding the dynamics of gene flow is essential for comprehending the complex mechanisms driving the evolution and diversification of species.
Question 6:
What evidence supports the theory of common descent, and how do homologous structures contribute to this evidence?
Answer:
Theory of Common Descent Defined:
The theory of common descent posits that all living organisms on Earth are related through descent from a common ancestor. This fundamental concept of evolutionary biology suggests that species diversify over time through processes like mutation, natural selection, and genetic drift.
Evidence Supporting Common Descent:
Homologous Structures:
- Definition: Anatomical features in different species that share a common origin but may serve different functions.
- Significance: Indicate evolutionary relationships and divergence from a common ancestor.
- Examples:
- Forelimbs of Vertebrates: The wings of bats, the arms of humans, the flippers of whales, and the legs of cats all have similar bone structures (humerus, radius, ulna, carpals, metacarpals, phalanges), suggesting a common ancestral forelimb.
Genetic Evidence:
- DNA Sequencing: Similarities in DNA sequences among different species indicate shared ancestry.
- Universal Genetic Code: The presence of the same genetic code across all known life forms suggests a common origin.
- Gene Homology: Genes that are conserved across species (homologous genes) point to shared ancestry.
Fossil Record:
- Transitional Fossils: Fossils showing intermediate forms between major groups provide evidence of evolutionary transitions.
- Example: Archaeopteryx exhibits features of both dinosaurs and modern birds, illustrating a transition from non-avian dinosaurs to birds.
- Chronological Succession: The order of fossils in geological strata supports the pattern of diversification from common ancestors.
Embryology:
- Similar Embryonic Stages: Embryos of different vertebrates display similar developmental stages, indicating common ancestry.
- Example: Early embryos of fish, birds, and mammals show similar structures like pharyngeal pouches and tails, which later differentiate into diverse anatomical features.
Biogeography:
- Geographical Distribution: The distribution of species across different continents and islands supports common descent and subsequent diversification.
- Example: The unique species found on the Galápagos Islands reflect evolutionary divergence from common mainland ancestors due to geographic isolation.
Vestigial Structures:
- Definition: Anatomical features that have lost their original function through evolution.
- Significance: Indicate remnants of structures present in ancestral species.
- Examples:
- Human Appendix: A vestigial structure thought to be a remnant of a larger cecum used by ancestral herbivorous humans for digesting cellulose.
- Pelvic Bones in Whales: Remnants of hind limbs from their terrestrial ancestors.
Molecular Biology:
- Protein and RNA Similarities: Conservation of protein sequences and RNA structures among different species.
- Mitochondrial DNA: Similarities in mitochondrial genomes across species support common descent.
Homologous Structures and Common Descent:
Homologous structures are pivotal evidence for common descent as they demonstrate how different organisms have inherited similar anatomical features from a shared ancestor. Despite serving different functions in various species, the underlying structural similarities reveal evolutionary relationships and the divergence of species from common ancestral forms.
Examples of Homologous Structures:
Vertebrate Limbs:
- Common Structure: Bones like the humerus, radius, and ulna are present in the forelimbs of mammals, birds, reptiles, and amphibians.
- Divergent Functions: While humans use their forelimbs for manipulation, birds use theirs for flying, and whales use theirs for swimming.
Flippers of Marine Mammals:
- Common Structure: Flippers of dolphins, seals, and manatees share similar bone structures derived from ancestral limbs.
- Divergent Functions: Adapted for efficient movement in water, demonstrating modification from their terrestrial origins.
Hind Limbs of Vertebrates:
- Common Structure: Pelvic bones in humans, birds, and whales indicate a common origin.
- Divergent Functions: Humans use them for locomotion on land, birds for perching or balance, and whales for swimming.
Conclusion:
The theory of common descent is strongly supported by multiple lines of evidence, with homologous structures providing compelling anatomical proof of shared ancestry among diverse organisms. These structures highlight the evolutionary pathways that have led to the vast diversity of life observed today, reinforcing the interconnectedness of all living species through common origins.
Question 7:
How do vestigial structures provide evidence for evolution, and can you provide examples from different organisms?
Answer:
Vestigial Structures Defined:
Vestigial structures are anatomical features that have lost most or all of their original function through the course of evolution. These structures are remnants of organs or parts that were functional in ancestral species but have become reduced or obsolete in descendants.
Evidence for Evolution Provided by Vestigial Structures:
Ancestral Origins: Vestigial structures indicate that an organism has evolved from ancestors with fully functional versions of these structures.
Evolutionary Modification: The presence of vestigial structures demonstrates how organisms can undergo structural changes over time, adapting to new environments and lifestyles.
Predictive Power: The existence of vestigial structures allows scientists to make predictions about the evolutionary history of organisms and verify them through comparative anatomy and the fossil record.
Examples of Vestigial Structures in Different Organisms:
Humans:
- Appendix: Once part of the digestive system for fermenting cellulose in ancestral herbivores, the human appendix is now largely redundant, though it may have minor immune functions.
- Wisdom Teeth: Evolved for grinding plant material in ancestors with larger jaws, but often impacted due to reduced jaw size in modern humans.
- Tailbone (Coccyx): The remnant of a lost tail, serving as an anchor point for muscles and ligaments in the pelvic region.
Whales:
- Pelvic Bones: Remnants of hind limbs from their terrestrial ancestors, no longer used for walking but serving as anchor points for reproductive organs.
- Hearing Structures: Vestigial ear bones that no longer play a role in terrestrial hearing but are part of the whale’s auditory system adapted for underwater acoustics.
Birds:
- Wing Claws: Present in some bird species like the hoatzin chicks, vestigial in most adult birds, indicating ancestral climbing abilities.
- Pelvic Bones: Reduced in size and function, reflecting the transition from ground-dwelling to primarily flying lifestyles.
Snakes:
- Hind Limb Remnants: Vestigial pelvic spurs in some snake species like boas and pythons, remnants of their limbed ancestors, used for mating.
- Spurs: Small, claw-like structures near the cloaca, vestigial limbs that no longer serve a locomotor function.
Frogs:
- Tail in Adult Frogs: Some species retain a tadpole-like tail during their larval stage, but it disappears as they metamorphose into adults, leaving behind no functional tail.
Flightless Birds:
- Wings in Ostriches and Emus: Present but incapable of flight, serving other functions such as balance or display.
- Flight Muscles: Reduced in size due to the lack of need for powered flight.
Insects:
- Wings in Some Ant Species: Worker ants in certain species have vestigial wings, remnants from their reproductive or ancestral forms.
- Eyes in Cave-Dwelling Species: Some cave insects have reduced or non-functional eyes, reflecting their adaptation to dark environments where vision is unnecessary.
Significance of Vestigial Structures in Understanding Evolution:
Demonstrate Ancestral Traits: Highlight features that were functional in ancestors, providing a link between present-day species and their evolutionary history.
Show Evolutionary Change: Illustrate how species adapt and modify structures over time in response to changing environments and lifestyles.
Support Common Descent: Provide anatomical evidence that different species share common ancestors, reinforcing the interconnectedness of life through evolution.
Conclusion:
Vestigial structures are powerful evidence for the theory of evolution, showcasing the remnants of ancestral organs that have lost their original functions. These structures across various organisms highlight the dynamic nature of evolution, demonstrating how species adapt, diversify, and sometimes lose traits as they evolve to fit new ecological niches.
Question 8:
What is the role of genetic mutations in evolution, and how can they lead to the development of new traits within a population?
Answer:
Genetic Mutations Defined:
Genetic mutations are changes in the DNA sequence of an organism’s genome. They can occur spontaneously during DNA replication or be induced by environmental factors such as radiation, chemicals, or viruses.
Role of Genetic Mutations in Evolution:
Source of Genetic Variation:
- Introducing New Alleles: Mutations create new alleles, contributing to the genetic diversity within a population.
- Allelic Diversity: Increased genetic variation provides raw material for natural selection to act upon, facilitating adaptation and evolutionary change.
Driving Force for Evolutionary Change:
- Novel Traits: Mutations can result in new or altered traits that may enhance an organism’s fitness or reduce it, depending on the environment.
- Adaptive Evolution: Beneficial mutations increase an organism’s chances of survival and reproduction, spreading advantageous traits through the population.
Facilitating Speciation:
- Genetic Divergence: Accumulation of mutations in isolated populations can lead to genetic divergence, potentially resulting in the formation of new species.
- Reproductive Isolation: Mutations that affect mating behaviors or reproductive compatibility can contribute to reproductive isolation, a key factor in speciation.
Types of Genetic Mutations:
Point Mutations:
- Substitutions: Replacement of one nucleotide with another (e.g., missense, nonsense mutations).
- Impact: Can alter protein function, sometimes leading to new traits or diseases.
Insertions and Deletions (Indels):
- Addition or Removal of Nucleotides: Can cause frameshift mutations, altering the reading frame of genes.
- Impact: Often leads to nonfunctional proteins, but can occasionally create novel protein functions.
Duplication Mutations:
- Replication of Genetic Material: Result in multiple copies of a gene or genomic region.
- Impact: Provides genetic redundancy, allowing one copy to acquire new functions without detrimental effects on the organism.
Inversion and Translocation Mutations:
- Chromosomal Rearrangements: Sections of chromosomes are flipped (inversion) or moved to different locations (translocation).
- Impact: Can disrupt gene function or create new gene combinations, potentially leading to new traits.
Mechanisms by Which Mutations Lead to New Traits:
Gene Expression Changes:
- Regulatory Mutations: Alterations in regulatory regions (promoters, enhancers) can change when, where, and how much a gene is expressed, leading to variations in traits.
- Example: Changes in regulatory genes can result in different flower colors or sizes.
Protein Function Alterations:
- Amino Acid Changes: Mutations can change the amino acid sequence of proteins, affecting their structure and function.
- Example: A single amino acid change in hemoglobin can lead to sickle cell anemia, illustrating how mutations can have significant phenotypic effects.
Gene Duplication and Divergence:
- Functional Redundancy: Duplication allows one gene copy to maintain its original function while the other is free to accumulate mutations that may lead to new functions.
- Example: The evolution of globin genes, where gene duplication led to the development of different hemoglobin types for oxygen transport in various tissues.
Loss of Function Mutations:
- Gene Inactivation: Mutations can render genes nonfunctional, leading to the loss of certain traits.
- Example: Vestigial structures, such as the human appendix, result from mutations that have inactivated their original functions.
Gain of Function Mutations:
- New Functions: Mutations can confer new abilities or enhance existing ones, contributing to adaptive traits.
- Example: The development of antibiotic resistance in bacteria through mutations that alter target sites or increase efflux pump activity.
Impact of Mutations on Population Genetics:
Allele Frequency Changes: Mutations introduce new alleles, altering allele frequencies within a population over time.
Evolutionary Potential: Higher mutation rates can increase the evolutionary potential of a population, enabling rapid adaptation to changing environments.
Genetic Load: Accumulation of deleterious mutations can negatively impact population fitness, but mechanisms like purifying selection help remove harmful alleles.
Conclusion:
Genetic mutations are essential drivers of evolutionary change, introducing the genetic diversity necessary for populations to adapt and evolve. By generating new alleles and altering existing ones, mutations facilitate the development of novel traits, contribute to speciation, and enhance the adaptive potential of organisms. Understanding the role of mutations in evolution is fundamental to comprehending the mechanisms underlying the diversity of life on Earth.
Question 9:
How does sexual selection differ from natural selection, and what are some examples of traits that have evolved primarily through sexual selection?
Answer:
Sexual Selection Defined:
Sexual selection is a mode of natural selection where certain traits increase an individual’s chances of successfully mating and reproducing. It arises from the differential reproductive success based on traits that are favored by the opposite sex or by intrasexual competition.
Differences Between Sexual Selection and Natural Selection:
Selection Pressure:
- Natural Selection: Driven by survival-related traits that enhance an organism’s ability to survive in its environment (e.g., camouflage, efficient metabolism).
- Sexual Selection: Driven by traits that enhance an organism’s ability to obtain mates, which may not necessarily improve survival and can sometimes even be detrimental.
Types of Traits Selected:
- Natural Selection: Traits that improve an organism’s fitness by increasing survival and overall reproductive success.
- Sexual Selection: Traits that increase mating success, such as elaborate ornaments, displays, or behaviors, even if they incur a survival cost.
Mechanisms:
- Natural Selection: Involves both interspecific and intraspecific interactions, focusing on traits that confer a survival advantage.
- Sexual Selection: Primarily involves intraspecific interactions, focusing on mate choice and competition for mates.
Types of Sexual Selection:
Intersexual Selection (Mate Choice):
- Definition: Selection where one sex (usually females) chooses mates based on certain desirable traits.
- Outcome: Traits preferred by the selecting sex become more prevalent in the population.
- Example: Female peacocks choosing males with extravagant tail feathers.
Intrasexual Selection (Competition):
- Definition: Selection where members of the same sex (usually males) compete with each other for access to mates.
- Outcome: Traits that enhance competitive abilities become more prevalent.
- Example: Male deer developing large antlers to fight rivals for access to females.
Examples of Traits Evolved Primarily Through Sexual Selection:
Peacock’s Tail:
- Description: Male peacocks possess large, colorful tail feathers used in courtship displays.
- Function: The elaborate tails attract female peahens, signaling the male’s fitness.
- Evolutionary Impact: Despite being cumbersome and energy-intensive to maintain, the tails persist because they increase mating success.
Lion’s Mane:
- Description: Male lions have prominent manes surrounding their heads.
- Function: The mane serves as a display of strength and virility, deterring rivals and attracting females.
- Evolutionary Impact: Males with fuller manes are more likely to secure mates and protect the pride, enhancing reproductive success.
Gorilla’s Chest Pouch:
- Description: Male gorillas have large chest pouches.
- Function: The pouch emphasizes their muscular build, signaling dominance and fitness to females and rival males.
- Evolutionary Impact: Males with larger pouches are more successful in mating and defending their group.
Hummingbird’s Iridescent Plumage:
- Description: Male hummingbirds display bright, iridescent feathers.
- Function: The vibrant colors attract females and indicate the male’s health and genetic quality.
- Evolutionary Impact: Males with more striking plumage have higher mating success, promoting the spread of these traits.
Elephant Seal’s Size:
- Description: Male elephant seals are significantly larger than females.
- Function: Larger size allows males to dominate territories and compete effectively for access to females.
- Evolutionary Impact: Males with greater size and strength secure more breeding opportunities, driving the evolution of gigantism in the species.
Bird-of-Paradise’s Elaborate Displays:
- Description: Male birds-of-paradise perform intricate dances and display vibrant feathers.
- Function: These displays attract females by showcasing the male’s vitality and genetic fitness.
- Evolutionary Impact: The complexity and beauty of the displays are favored, leading to the diversification of species with unique ornamental traits.
Stallion’s Mane and Behavior:
- Description: Stallions exhibit prominent manes and aggressive behaviors during mating seasons.
- Function: The mane and behavior signal dominance and attractiveness to mares.
- Evolutionary Impact: Stallions with more impressive manes and assertive behaviors have higher reproductive success.
Consequences of Sexual Selection:
Exaggerated Traits: Traits favored by sexual selection can become highly exaggerated, sometimes at the expense of survival.
Sexual Dimorphism: Significant differences in appearance or behavior between males and females of a species often result from sexual selection.
Speciation: Divergent sexual preferences can lead to reproductive isolation and eventually speciation.
Conclusion:
Sexual selection is a powerful evolutionary force that shapes the diversity of traits observed in the animal kingdom. By favoring traits that enhance mating success, sexual selection drives the development of elaborate and often costly features, contributing to the rich tapestry of life’s morphological and behavioral diversity.
Question 10:
What mechanisms underlie the development of antibiotic resistance in bacteria, and how does this illustrate the principles of evolution?
Answer:
Development of Antibiotic Resistance Defined:
Antibiotic resistance in bacteria is the ability of bacteria to survive and proliferate despite the presence of antibiotics that would typically inhibit or kill them. This phenomenon poses significant challenges to public health by rendering standard treatments ineffective.
Mechanisms Underlying Antibiotic Resistance:
Genetic Mutations:
- Spontaneous Mutations: Random changes in the bacterial DNA can lead to alterations in target sites of antibiotics, reducing the drug’s efficacy.
- Example: Mutations in the gene encoding DNA gyrase can confer resistance to quinolone antibiotics.
Horizontal Gene Transfer (HGT):
- Transformation: Uptake of free DNA fragments from the environment by competent bacteria.
- Transduction: Transfer of genetic material between bacteria via bacteriophages (viruses that infect bacteria).
- Conjugation: Direct transfer of plasmids (small, circular DNA molecules) carrying resistance genes between bacteria through cell-to-cell contact.
- Impact: Facilitates rapid spread of resistance genes across bacterial populations and species.
Efflux Pumps:
- Mechanism: Proteins that actively expel antibiotics from bacterial cells, reducing intracellular drug concentration.
- Example: The AcrAB-TolC efflux pump in Escherichia coli confers resistance to multiple antibiotics by pumping them out of the cell.
Enzymatic Degradation:
- Mechanism: Production of enzymes that inactivate antibiotics by breaking them down or modifying their structure.
- Example: Bacillus cereus produces beta-lactamase enzymes that hydrolyze the beta-lactam ring in penicillin, rendering it ineffective.
Target Modification:
- Mechanism: Alteration of antibiotic binding sites on bacterial proteins, preventing the drug from effectively targeting its intended site.
- Example: Modification of ribosomal proteins in Staphylococcus aureus leads to resistance to macrolide antibiotics.
Reduced Permeability:
- Mechanism: Changes in the bacterial cell membrane or wall that decrease antibiotic entry into the cell.
- Example: Alterations in porin channels in Klebsiella pneumoniae reduce the uptake of beta-lactam antibiotics.
Illustration of Evolutionary Principles:
Variation:
- Genetic Diversity: Bacterial populations harbor genetic variations, including mutations and acquired resistance genes, providing the raw material for evolution.
Inheritance:
- Heritable Traits: Resistance traits, whether through mutations or gene acquisition, are heritable and can be passed to subsequent generations.
Differential Survival and Reproduction:
- Selective Pressure: The presence of antibiotics creates a selective environment where resistant bacteria have a survival and reproductive advantage over susceptible ones.
Adaptation:
- Increased Resistance: Over time, the frequency of resistance alleles increases in the population, leading to a predominantly resistant bacterial community.
Evolutionary Change:
- Population Shift: The bacterial population evolves to become more resistant to antibiotics, demonstrating evolutionary change in response to environmental pressures.
Consequences of Antibiotic Resistance:
- Treatment Challenges:
- Increased Morbidity and Mortality: Resistant infections are harder to treat, leading to longer illnesses and higher death rates.
- Economic Impact:
- Healthcare Costs: Increased expenses due to prolonged hospital stays, additional diagnostic tests, and the use of more expensive or toxic drugs.
- Public Health Threats:
- Spread of Resistance: Resistant bacteria can spread within communities and healthcare settings, exacerbating the problem.
Strategies to Combat Antibiotic Resistance:
- Antibiotic Stewardship:
- Rational Use: Prescribing antibiotics only when necessary and appropriate to minimize selective pressure.
- Infection Control:
- Preventing Spread: Implementing hygiene measures, isolation protocols, and vaccination to reduce transmission of resistant bacteria.
- Research and Development:
- New Antibiotics: Investing in the discovery of novel antibiotics and alternative therapies to overcome resistance mechanisms.
- Public Education:
- Awareness Campaigns: Educating the public about the responsible use of antibiotics and the dangers of misuse.
Conclusion:
The development of antibiotic resistance in bacteria exemplifies the principles of evolution, demonstrating how genetic variation and selective pressures drive evolutionary change. Understanding the mechanisms behind resistance and implementing comprehensive strategies to mitigate its spread are crucial for maintaining the efficacy of antibiotics and safeguarding public health.
Question 11:
How do homologous and analogous structures differ, and what do they reveal about the evolutionary relationships between organisms?
Answer:
Homologous Structures Defined:
Homologous structures are anatomical features in different species that share a common evolutionary origin but may serve different functions. These structures arise from a common ancestor and exhibit similar underlying anatomy despite divergent uses.
Examples of Homologous Structures:
Vertebrate Limbs:
- Structure: The forelimbs of mammals, birds, reptiles, and amphibians share a similar bone arrangement (humerus, radius, ulna, carpals, metacarpals, phalanges).
- Function: Despite the common structure, the limbs have adapted to different functions such as flying in birds, swimming in whales, and grasping in primates.
Pentadactyl Limb:
- Definition: A limb with five digits, a characteristic of all tetrapods.
- Significance: Indicates a shared ancestor from which all tetrapods descended.
Analogous Structures Defined:
Analogous structures are anatomical features in different species that serve similar functions but do not share a common evolutionary origin. These structures arise through convergent evolution, where unrelated species independently evolve similar traits to adapt to similar environments or ecological niches.
Examples of Analogous Structures:
Wings of Bats and Insects:
- Function: Enable flight.
- Structure: Despite serving the same function, bat wings are modified forelimbs with a membrane stretched over elongated fingers, while insect wings are extensions of the exoskeleton.
Eyes of Octopuses and Humans:
- Function: Vision.
- Structure: Both have camera-type eyes, but their anatomical development is distinct, reflecting independent evolutionary paths.
Differences Between Homologous and Analogous Structures:
Evolutionary Origin:
- Homologous Structures: Share a common ancestor.
- Analogous Structures: Do not share a common ancestor; similarity arises from similar selective pressures.
Underlying Anatomy:
- Homologous Structures: Exhibit similar bone structures, developmental pathways, and genetic underpinnings.
- Analogous Structures: Have different anatomical compositions and developmental origins despite similar functions.
Evolutionary Significance:
- Homologous Structures: Provide evidence for common descent and evolutionary relationships between species.
- Analogous Structures: Illustrate convergent evolution and the adaptive responses of unrelated species to similar environmental challenges.
Implications for Understanding Evolutionary Relationships:
Homologous Structures:
- Phylogenetic Insights: Help construct evolutionary trees by revealing shared ancestry.
- Evolutionary Constraints: Highlight how organisms can modify existing structures for new functions without altering the fundamental anatomical framework.
Analogous Structures:
- Ecological Adaptations: Demonstrate how similar environmental pressures can lead to similar functional adaptations in unrelated species.
- Convergent Evolution: Show the power of natural selection in shaping similar solutions to comparable ecological challenges.
Case Studies Illustrating the Differences:
Homologous vs. Analogous Wings:
- Bats (Homologous): Wings evolved from forelimbs with a common skeletal structure.
- Insects (Analogous): Wings evolved independently from the exoskeleton without a shared skeletal origin.
Homologous vs. Analogous Eyes:
- Humans (Homologous): Eyes share a common structural blueprint with other vertebrates.
- Cephalopods (Analogous): Eyes evolved independently with a similar camera-like function but different structural development.
Conclusion:
Homologous and analogous structures provide crucial insights into the evolutionary history and relationships between organisms. Homologous structures underscore common ancestry and shared evolutionary pathways, while analogous structures highlight the role of convergent evolution in shaping similar adaptations among unrelated species. Distinguishing between these types of structures is essential for understanding the complexities of evolutionary biology and the diverse strategies life employs to thrive in various environments.
Question 12:
What is the significance of the fossil record in understanding evolutionary history, and what are some limitations of fossil data?
Answer:
Fossil Record Defined:
The fossil record is the collection of all known fossils and their placement in rock formations and sedimentary layers. It provides a historical record of life on Earth, documenting the existence, diversity, and changes of organisms over geological time.
Significance of the Fossil Record in Understanding Evolutionary History:
Documentation of Past Life:
- Historical Evidence: Fossils provide direct evidence of organisms that lived in the past, offering insights into their morphology, behavior, and ecology.
Chronological Framework:
- Geological Time Scale: Fossils are found in specific rock layers, allowing scientists to establish the relative ages of different organisms and trace the timeline of evolutionary events.
Transitional Forms:
- Evolutionary Linkages: Fossils of transitional species demonstrate the gradual changes between major groups, supporting the theory of common descent.
- Example: Archaeopteryx exhibits features of both non-avian dinosaurs and modern birds, illustrating the transition from dinosaurs to birds.
Patterns of Evolution:
- Adaptive Radiation: Fossil evidence shows periods of rapid diversification, such as the Cambrian Explosion, where many major animal phyla first appear.
- Mass Extinctions: Fossils reveal patterns of mass extinctions and subsequent recoveries, shaping the course of evolution by eliminating dominant groups and allowing new ones to emerge.
Biogeography:
- Distribution Patterns: Fossils help trace the historical distribution of species across continents, providing evidence for plate tectonics and continental drift.
- Example: Similar fossils found on now-separated continents support the idea of ancient land connections.
Environmental and Climatic Changes:
- Paleoecology: Fossils indicate past environmental conditions and how organisms adapted to changes, informing our understanding of ecological and climatic shifts.
- Example: Fossils of tropical plants in polar regions suggest past global temperature variations.
Limitations of Fossil Data:
Incomplete Record:
- Preservation Bias: Not all organisms are equally likely to be fossilized. Hard-bodied organisms (e.g., bones, shells) are more commonly preserved than soft-bodied ones.
- Rare Conditions: Fossilization requires specific conditions (rapid burial, low oxygen) that are not always present, leading to gaps in the record.
Temporal Gaps:
- Missing Intervals: Large spans of geological time may lack fossil evidence due to erosion, non-deposition, or destruction of rock layers.
Geographical Limitations:
- Limited Access: Fossils are often found in specific regions, which may not represent global biodiversity or the full range of past life forms.
Identification Challenges:
- Fragmentary Fossils: Many fossils are incomplete or fragmented, making accurate identification and classification difficult.
Interpretation Ambiguities:
- Convergent Evolution: Similar fossil features may arise from convergent evolution, complicating interpretations of evolutionary relationships.
Dating Uncertainties:
- Relative Dating: Fossils are often dated relative to other fossils or rock layers, but absolute dating methods can have margins of error.
- Radiometric Limitations: Certain radiometric dating techniques are only applicable to specific types of rocks and minerals.
Bias Toward Certain Environments:
- Marine vs. Terrestrial: Marine environments are more conducive to fossil preservation, leading to a richer marine fossil record compared to terrestrial ecosystems.
Examples Highlighting Limitations:
Soft-Bodied Organisms: The Burgess Shale fossils are exceptional for preserving soft-bodied organisms, but such preservation is rare, limiting our understanding of most soft-bodied life forms.
Gaps in the Mesozoic Era: While the fossil record includes many dinosaur fossils, gaps still exist in certain periods and regions, hindering a complete picture of their evolution and extinction.
Advancements to Overcome Limitations:
- Technological Innovations:
- Imaging Techniques: Advanced imaging methods (e.g., CT scanning) allow for detailed analysis of fossil structures without damaging specimens.
- Enhanced Dating Methods:
- Improved Radiometric Techniques: Refinements in dating methods increase accuracy and precision in determining fossil ages.
- Global Collaboration:
- International Research: Collaborative efforts expand fossil discoveries across different regions, enriching the overall fossil record.
- Interdisciplinary Approaches:
- Integrating Data: Combining fossil evidence with genetic, developmental, and ecological data provides a more comprehensive understanding of evolutionary history.
Conclusion:
The fossil record is an invaluable resource for unraveling the history of life on Earth, offering direct evidence of past organisms and their evolutionary transitions. Despite its limitations, ongoing scientific advancements and collaborative research continue to enhance our understanding of evolutionary processes. By addressing gaps and biases, the fossil record remains a cornerstone of evolutionary biology, illuminating the dynamic and interconnected nature of life through geological time.
Evolutionary Biology: Thought-Provoking Questions
1. How does natural selection drive the evolution of specific traits within a population, and what are some real-world examples of this process?
Answer:
Natural Selection Defined:
Natural selection is a fundamental mechanism of evolution proposed by Charles Darwin. It describes how traits that enhance an organism’s survival and reproductive success become more common in successive generations of a population.
Key Components of Natural Selection:
- Variation: Individuals within a population exhibit variations in their traits (e.g., size, coloration, speed).
- Inheritance: Some of these traits are heritable and can be passed from parents to offspring.
- Differential Survival and Reproduction: Individuals with advantageous traits are more likely to survive and reproduce, passing those traits to the next generation.
- Adaptation: Over time, beneficial traits become more prevalent, leading to populations better suited to their environments.
Mechanisms of Natural Selection:
- Directional Selection: Favors one extreme trait over the mean or other extreme. Example: The increase in antibiotic-resistant bacteria.
- Stabilizing Selection: Favors the average trait, reducing variation. Example: Human birth weights, where extremely low or high weights have higher mortality rates.
- Disruptive Selection: Favors extreme traits at both ends, increasing variation. Example: Beak sizes in certain bird species where both very large and very small beaks are advantageous.
Real-World Examples:
Peppered Moth (Biston betularia):
- Before Industrialization: Light-colored moths were common as they blended with lichen-covered trees.
- After Industrialization: Pollution darkened the trees, favoring dark-colored moths that were less visible to predators.
- Result: Dark moths became more prevalent in polluted areas, demonstrating natural selection in response to environmental change.
Darwin’s Finches:
- Variation: Finches on the Galápagos Islands exhibit a range of beak shapes and sizes.
- Selection Pressure: Availability of different food sources, such as seeds, insects, and flowers.
- Result: Finches with beak shapes suited to available food sources had higher survival and reproductive success, leading to diversification of finch species.
Antibiotic Resistance in Bacteria:
- Variation: Some bacteria possess mutations that confer resistance to antibiotics.
- Selection Pressure: Use of antibiotics kills susceptible bacteria, while resistant ones survive.
- Result: Resistant bacteria proliferate, making infections harder to treat.
Industrial Melanism in the Scottish Wild Rabbit:
- Before Fur Dyeing: Predators hunted rabbits that stood out against the light-colored ground.
- After Fur Dyeing: Dark-colored rabbits had a survival advantage as they were better camouflaged.
- Result: Increase in the frequency of dark-colored rabbits in affected areas.
Implications of Natural Selection:
- Adaptation: Populations become better suited to their environments over time.
- Speciation: Accumulation of advantageous traits can lead to the formation of new species.
- Biodiversity: Natural selection contributes to the diversity of life by promoting a variety of adaptations.
Conclusion:
Natural selection is a powerful force shaping the evolution of species. By favoring traits that enhance survival and reproduction, it drives populations to adapt to their environments, leading to the rich diversity of life observed today. Real-world examples like the peppered moth, Darwin’s finches, and antibiotic-resistant bacteria vividly illustrate how natural selection operates in nature.
2. What evidence supports the theory of common descent, and how do homologous structures contribute to this evidence?
Answer:
Theory of Common Descent Defined:
The theory of common descent posits that all living organisms on Earth share a common ancestor. This foundational concept of evolutionary biology suggests that species diverge from shared lineages over time through processes like mutation, natural selection, and genetic drift.
Evidence Supporting Common Descent:
Homologous Structures:
- Definition: Anatomical features in different species that share a common origin but may serve different functions.
- Significance: Indicate evolutionary relationships and divergence from a common ancestor.
- Examples:
- Vertebrate Limbs: The forelimbs of mammals, birds, reptiles, and amphibians share a similar bone structure (humerus, radius, ulna, carpals, metacarpals, phalanges) despite serving different functions like grasping, flying, swimming, and walking.
- Pentadactyl Limb: A limb with five digits, characteristic of all tetrapods, suggesting a common ancestral limb structure.
Genetic Evidence:
- DNA Sequencing: Similarities in DNA sequences among different species indicate shared ancestry.
- Universal Genetic Code: The presence of the same genetic code across all known life forms suggests a common origin.
- Gene Homology: Genes that are conserved across species (homologous genes) point to shared ancestry.
Fossil Record:
- Transitional Fossils: Fossils showing intermediate forms between major groups provide evidence of evolutionary transitions.
- Example: Archaeopteryx exhibits features of both non-avian dinosaurs and modern birds, illustrating the transition from dinosaurs to birds.
- Chronological Succession: The order of fossils in geological strata supports the pattern of diversification from common ancestors.
Embryology:
- Similar Embryonic Stages: Embryos of different vertebrates display similar developmental stages, indicating common ancestry.
- Example: Early embryos of fish, birds, and mammals show similar structures like pharyngeal pouches and tails, which later differentiate into diverse anatomical features.
Biogeography:
- Geographical Distribution: The distribution of species across different continents and islands supports common descent and subsequent diversification.
- Example: The unique species found on the Galápagos Islands reflect evolutionary divergence from common mainland ancestors due to geographic isolation.
Vestigial Structures:
- Definition: Anatomical features that have lost their original function through evolution.
- Examples:
- Human Appendix: A vestigial structure thought to be a remnant of a larger cecum used by ancestral herbivorous humans for digesting cellulose.
- Pelvic Bones in Whales: Remnants of hind limbs from their terrestrial ancestors.
Molecular Biology:
- Protein and RNA Similarities: Conservation of protein sequences and RNA structures among different species.
- Mitochondrial DNA: Similarities in mitochondrial genomes across species support common descent.
Homologous Structures and Common Descent:
Homologous structures are pivotal evidence for common descent as they demonstrate how different organisms have inherited similar anatomical features from a shared ancestor. Despite serving different functions in various species, the underlying structural similarities reveal evolutionary relationships and the divergence of species from common ancestral forms.
Examples of Homologous Structures:
Vertebrate Limbs:
- Common Structure: The forelimbs of mammals, birds, reptiles, and amphibians share a similar bone arrangement.
- Divergent Functions: While humans use their forelimbs for manipulation, birds use theirs for flying, and whales use theirs for swimming.
Flippers of Marine Mammals:
- Common Structure: Flippers of dolphins, seals, and manatees share similar bone structures derived from ancestral limbs.
- Divergent Functions: Adapted for efficient movement in water, demonstrating modification from their terrestrial origins.
Hind Limbs of Vertebrates:
- Common Structure: Pelvic bones in humans, birds, and whales indicate a common origin.
- Divergent Functions: Humans use them for locomotion on land, birds for perching or balance, and whales for swimming.
Conclusion:
The theory of common descent is strongly supported by multiple lines of evidence, with homologous structures providing compelling anatomical proof of shared ancestry among diverse organisms. These structures highlight the evolutionary pathways that have led to the vast diversity of life observed today, reinforcing the interconnectedness of all living species through common origins.
3. How does genetic drift differ from natural selection, and what role does it play in the evolution of small populations?
Answer:
Genetic Drift Defined:
Genetic drift is a mechanism of evolution that involves random changes in allele frequencies within a population. Unlike natural selection, which is driven by environmental pressures and differential fitness, genetic drift occurs by chance and can significantly impact small populations.
Differences Between Genetic Drift and Natural Selection:
Mechanism:
- Genetic Drift: Driven by random chance events that alter allele frequencies, independent of allele advantages or disadvantages.
- Natural Selection: Driven by differential survival and reproduction based on alleles that confer a fitness advantage or disadvantage.
Directionality:
- Genetic Drift: Changes in allele frequencies are random and can increase or decrease alleles regardless of their impact on fitness.
- Natural Selection: Changes in allele frequencies are non-random and typically favor alleles that enhance fitness.
Impact on Genetic Variation:
- Genetic Drift: Can reduce genetic variation by randomly eliminating alleles, especially in small populations.
- Natural Selection: Can either increase genetic variation by favoring diverse advantageous traits or reduce it by eliminating deleterious alleles.
Population Size Sensitivity:
- Genetic Drift: More pronounced in small populations where random events can lead to significant changes in allele frequencies.
- Natural Selection: Operates in populations of all sizes but has a more substantial influence in larger populations where selection pressures consistently favor certain alleles.
Role of Genetic Drift in the Evolution of Small Populations:
Allele Fixation and Loss:
- Fixation: An allele becomes fixed (frequency reaches 100%) purely by chance.
- Loss: An allele can be lost from the population without regard to its effect on fitness.
Bottleneck Effect:
- Definition: Occurs when a population undergoes a drastic reduction in size due to events like natural disasters, leading to a loss of genetic diversity.
- Impact: The surviving population may have different allele frequencies than the original population, potentially reducing genetic variation.
Founder Effect:
- Definition: Happens when a new population is established by a small number of individuals from a larger population.
- Impact: The new population may have allele frequencies that differ from the original population, leading to reduced genetic diversity and potential for unique evolutionary paths.
Genetic Drift and Adaptation:
- Neutral Evolution: Genetic drift often acts on neutral alleles that do not affect an organism’s fitness, allowing them to spread or disappear randomly.
- Non-Neutral Evolution: While most drift affects neutral alleles, it can also influence slightly deleterious or advantageous alleles in small populations, potentially impacting adaptation.
Examples Illustrating Genetic Drift:
Northern Elephant Seals:
- History: Once reduced to as few as 20 individuals due to hunting.
- Current Status: Present populations have extremely low genetic diversity, a consequence of the bottleneck effect caused by genetic drift.
Galápagos Finches:
- Founder Effect: Colonization of new islands by a small number of finches led to unique species with distinct beak shapes adapted to local food sources.
Cheetahs:
- Low Genetic Diversity: Cheetah populations have experienced genetic bottlenecks, resulting in reduced genetic variation and increased susceptibility to diseases.
Implications of Genetic Drift:
- Vulnerability to Extinction: Reduced genetic diversity can make small populations more susceptible to extinction due to diseases, environmental changes, and inbreeding.
- Evolutionary Pathways: Genetic drift can lead to unique evolutionary trajectories in isolated small populations, contributing to biodiversity.
- Conservation Challenges: Managing small populations requires strategies to maintain genetic diversity and mitigate the effects of genetic drift.
Conclusion:
Genetic drift is a random evolutionary mechanism that plays a significant role in shaping the genetic structure of small populations. Unlike natural selection, which systematically favors advantageous traits, genetic drift can lead to unpredictable changes in allele frequencies, reducing genetic diversity and influencing the evolutionary path of populations. Understanding genetic drift is essential for conservation biology, particularly in managing endangered species and maintaining healthy, resilient populations.
4. What are the different modes of speciation, and how do they contribute to the formation of new species?
Answer:
Speciation Defined:
Speciation is the evolutionary process through which new distinct species arise from existing ones. It involves the divergence of populations to the point where they can no longer interbreed and produce fertile offspring.
Modes of Speciation:
Allopatric Speciation:
- Definition: Occurs when populations are geographically separated, leading to reproductive isolation.
- Process:
- Geographic Isolation: Physical barriers (mountains, rivers, distance) split a population into separate groups.
- Genetic Divergence: Isolated populations accumulate genetic differences through mutation, genetic drift, and natural selection.
- Reproductive Isolation: Over time, genetic and behavioral differences prevent interbreeding even if populations come into contact again.
- Example: The formation of the Grand Canyon led to the divergence of North American and Arizona populations of the Kaibab squirrel.
Sympatric Speciation:
- Definition: Occurs within a single geographic area without physical barriers, often driven by ecological, behavioral, or genetic factors.
- Process:
- Ecological Niches: Different groups exploit different resources or habitats within the same area.
- Behavioral Isolation: Changes in mating preferences or behaviors lead to reproductive isolation.
- Polyploidy (in plants): Duplication of the chromosome number creates reproductive barriers.
- Example: Apple maggot flies (Rhagoletis pomonella) shifted from hawthorn to apple trees, leading to reproductive isolation and speciation.
Peripatric Speciation:
- Definition: A form of allopatric speciation where a small peripheral population becomes isolated from the main population.
- Process:
- Founder Effect: A small group establishes a new population, leading to rapid genetic changes due to drift.
- Genetic Divergence: The isolated population evolves independently, accumulating unique genetic traits.
- Reproductive Isolation: Differences become significant enough to prevent interbreeding with the original population.
- Example: The Galápagos finches evolved into multiple species from a small ancestral population.
Parapatric Speciation:
- Definition: Occurs when populations are adjacent to each other with a narrow zone of overlap, leading to speciation without complete geographic isolation.
- Process:
- Environmental Gradients: Differences in environmental conditions across a continuous habitat create distinct selective pressures.
- Limited Gene Flow: Restricted interbreeding occurs at the boundary, allowing populations to diverge genetically.
- Reproductive Isolation: Over time, genetic differences lead to reproductive barriers.
- Example: Grasshopper species along different elevations in the same mountain range develop distinct traits adapted to their specific environments.
Hybrid Speciation:
- Definition: Occurs when two different species interbreed and produce hybrid offspring that are reproductively isolated from both parent species.
- Process:
- Hybridization: Interbreeding between two distinct species.
- Genetic Novelty: Hybrids possess a unique combination of traits from both parents.
- Reproductive Isolation: Hybrids are sterile or have reduced fertility, preventing gene flow between parent species.
- Example: The Italian sparrow is a hybrid of the house sparrow and the Spanish sparrow, forming a distinct species.
Mechanisms Facilitating Speciation:
- Genetic Drift: Random changes in allele frequencies, especially in small populations, can lead to reproductive isolation.
- Natural Selection: Divergent selection pressures in different environments can promote adaptation and genetic divergence.
- Mutation: Introduction of new genetic variations provides the raw material for speciation.
- Behavioral Changes: Changes in mating behaviors or preferences can lead to reproductive isolation.
- Polyploidy: Especially in plants, chromosome duplication can create reproductive barriers.
Implications of Speciation:
- Biodiversity: Speciation increases the number of species, contributing to the richness and complexity of ecosystems.
- Adaptation: New species can occupy different ecological niches, enhancing ecosystem resilience and functionality.
- Evolutionary Innovation: Speciation drives the emergence of novel traits and adaptations, fueling evolutionary progress.
Conclusion:
Speciation is a dynamic and multifaceted process essential for the diversification of life on Earth. Understanding the different modes of speciation—allopatric, sympatric, peripatric, parapatric, and hybrid—provides insights into how new species emerge and adapt, shaping the evolutionary landscape of biodiversity.
5. How do homologous structures provide evidence for common ancestry, and what distinguishes them from analogous structures?
Answer:
Homologous Structures Defined:
Homologous structures are anatomical features in different species that share a common evolutionary origin but may serve different functions. These structures arise from a common ancestor and exhibit similar underlying anatomy despite divergent uses.
Evidence for Common Ancestry:
Shared Anatomy:
- Bone Structure: Similar bone arrangements in the forelimbs of mammals, birds, reptiles, and amphibians indicate a shared ancestral limb structure.
- Example: The forelimbs of humans (arms), bats (wings), whales (flippers), and cats (legs) all contain the same basic bones arranged in similar patterns.
Developmental Pathways:
- Embryonic Similarities: Homologous structures often develop from the same embryonic tissues, reflecting their common origin.
- Example: During embryonic development, vertebrate limbs develop from limb buds that share similar developmental genes and pathways.
Genetic Evidence:
- Conserved Genes: Homologous structures are often associated with conserved genetic sequences that control their development.
- Example: The Hox genes, which play a crucial role in body plan development, are highly conserved across diverse animal species, correlating with the presence of homologous structures.
Fossil Record:
- Transitional Fossils: Fossils show intermediate forms that display homologous structures, bridging gaps between major groups.
- Example: Tiktaalik roseae exhibits features of both fish and tetrapods, demonstrating the transition of limb structures from aquatic to terrestrial environments.
Distinction from Analogous Structures:
Evolutionary Origin:
- Homologous Structures: Share a common ancestral origin.
- Analogous Structures: Do not share a common ancestral origin; similarities arise from convergent evolution due to similar selective pressures.
Underlying Anatomy:
- Homologous Structures: Have similar anatomical features and bone structures.
- Analogous Structures: Serve similar functions but have different anatomical structures.
- Example: The wings of birds (modified forelimbs with feathers) and the wings of insects (extensions of the exoskeleton) are analogous, not homologous.
Developmental Pathways:
- Homologous Structures: Develop from similar embryonic tissues and genetic pathways.
- Analogous Structures: Develop from different embryonic origins and genetic mechanisms.
Examples Highlighting the Differences:
Homologous Structures:
- Vertebrate Limbs: Similar bone structures across mammals, birds, reptiles, and amphibians.
- Pentadactyl Limb: A limb with five digits, found in all tetrapods, indicating a common ancestor.
Analogous Structures:
- Wings of Birds and Insects: Serve the same function (flight) but have different structural origins.
- Eyes of Octopuses and Humans: Both have camera-type eyes, but their anatomical development is distinct, reflecting independent evolutionary paths.
Implications for Understanding Evolution:
Phylogenetic Relationships:
- Homologous Structures: Help construct evolutionary trees by revealing shared ancestry.
- Analogous Structures: Highlight convergent evolution and the adaptive responses of unrelated species to similar environmental challenges.
Evolutionary Constraints and Flexibility:
- Homologous Structures: Show how organisms can modify existing structures for new functions without altering the fundamental anatomical framework.
- Analogous Structures: Demonstrate the versatility of natural selection in shaping similar functional solutions in different lineages.
Conclusion:
Homologous structures are compelling evidence for common ancestry, showcasing the shared evolutionary history among diverse organisms. By contrasting homologous structures with analogous ones, scientists can elucidate the pathways of evolutionary divergence and convergence, deepening our understanding of the interconnectedness of life on Earth.
6. What is genetic drift, and how does it differ from natural selection in shaping genetic variation within populations?
Answer:
Genetic Drift Defined:
Genetic drift is a mechanism of evolution that involves random changes in allele frequencies within a population. Unlike natural selection, which is driven by environmental pressures and differential fitness, genetic drift occurs by chance and can significantly impact small populations.
Key Characteristics of Genetic Drift:
- Random Sampling: Genetic drift results from random sampling of alleles during reproduction, where some alleles may be passed on more frequently purely by chance.
- Population Size: The effects of genetic drift are more pronounced in small populations because random events can lead to significant changes in allele frequencies.
- Allele Fixation and Loss: Over time, genetic drift can lead to the fixation (allele frequency reaches 100%) or complete loss (allele frequency reaches 0%) of alleles in a population.
Differences Between Genetic Drift and Natural Selection:
Mechanism:
- Genetic Drift: Driven by random chance without regard to the alleles’ effects on fitness.
- Natural Selection: Driven by differential survival and reproduction based on allele advantages or disadvantages.
Directionality:
- Genetic Drift: Changes in allele frequencies are random and can increase or decrease alleles regardless of their impact on fitness.
- Natural Selection: Changes in allele frequencies are non-random and typically favor alleles that enhance fitness.
Impact on Genetic Variation:
- Genetic Drift: Can reduce genetic variation by randomly eliminating alleles, especially in small populations.
- Natural Selection: Can either increase genetic variation by favoring diverse advantageous traits or reduce it by eliminating deleterious alleles.
Population Size Sensitivity:
- Genetic Drift: More significant in small populations where random events can drastically alter allele frequencies.
- Natural Selection: Operates in populations of all sizes but has a more substantial influence in larger populations where selection pressures consistently favor certain alleles.
Role of Genetic Drift in the Evolution of Small Populations:
Allele Fixation and Loss:
- Fixation: An allele becomes fixed (frequency reaches 100%) purely by chance.
- Loss: An allele can be lost from the population without regard to its effect on fitness.
Bottleneck Effect:
- Definition: Occurs when a population undergoes a drastic reduction in size due to events like natural disasters, leading to a loss of genetic diversity.
- Impact: The surviving population may have different allele frequencies than the original population, potentially reducing genetic variation.
Founder Effect:
- Definition: Happens when a new population is established by a small number of individuals from a larger population.
- Impact: The new population may have allele frequencies that differ from the original population, leading to reduced genetic diversity and potential for unique evolutionary paths.
Genetic Drift and Adaptation:
- Neutral Evolution: Genetic drift can drive changes in allele frequencies without any impact on fitness, particularly affecting neutral alleles.
- Non-Neutral Evolution: In small populations, genetic drift can also influence the frequencies of slightly deleterious or advantageous alleles, potentially impacting adaptation.
Examples Illustrating Genetic Drift:
Northern Elephant Seals:
- History: Once reduced to as few as 20 individuals due to hunting.
- Current Status: Present populations have extremely low genetic diversity, a consequence of the bottleneck effect caused by genetic drift.
Galápagos Finches:
- Founder Effect: Colonization of new islands by a small number of finches led to unique species with distinct beak shapes adapted to local food sources.
Cheetahs:
- Low Genetic Diversity: Cheetah populations have experienced genetic bottlenecks, resulting in reduced genetic variation and increased susceptibility to diseases.
Implications of Genetic Drift:
- Vulnerability to Extinction: Reduced genetic diversity can make small populations more susceptible to extinction due to diseases, environmental changes, and inbreeding.
- Evolutionary Pathways: Genetic drift can lead to unique evolutionary trajectories in isolated small populations, contributing to biodiversity.
- Conservation Challenges: Managing small populations requires strategies to maintain genetic diversity and mitigate the effects of genetic drift.
Conclusion:
Genetic drift is a random evolutionary mechanism that plays a significant role in shaping the genetic structure of small populations. Unlike natural selection, which systematically favors advantageous traits, genetic drift can lead to unpredictable changes in allele frequencies, reducing genetic diversity and influencing the evolutionary path of populations. Understanding genetic drift is essential for conservation biology, particularly in managing endangered species and maintaining healthy, resilient populations.
7. What role do gene flow and genetic exchange play in maintaining genetic diversity within populations, and how can they influence the process of speciation?
Answer:
Gene Flow Defined:
Gene flow, also known as gene migration, is the transfer of genetic material (alleles) between separate populations of the same species. It occurs when individuals from one population migrate to another, interbreed, and introduce new alleles into the gene pool.
Role of Gene Flow in Maintaining Genetic Diversity:
- Introduction of New Alleles:
- Allelic Variation: Gene flow introduces alleles that may be absent or rare in the recipient population, increasing genetic diversity.
- Reducing Genetic Differences:
- Homogenization: By mixing gene pools, gene flow reduces genetic differentiation between populations, promoting genetic similarity.
- Enhancing Adaptive Potential:
- Increased Variation: Higher genetic diversity provides a broader range of traits that may enhance a population’s ability to adapt to environmental changes.
Mechanisms Facilitating Gene Flow:
- Migration: Movement of individuals between populations through dispersal or migration.
- Hybridization: Interbreeding between different populations, often following secondary contact after geographic separation.
Impact of Gene Flow on Speciation:
Counteracting Speciation:
- Preventing Divergence: Gene flow can inhibit speciation by maintaining genetic similarity between populations, preventing the accumulation of genetic differences necessary for reproductive isolation.
- Example: Continuous migration between two populations can keep allele frequencies similar, reducing the likelihood of speciation.
Facilitating Speciation:
- Hybrid Vigor: In some cases, gene flow can introduce beneficial alleles that enhance fitness, promoting divergence if certain traits are selected.
- Adaptive Introgression: Gene flow can transfer advantageous genes from one population to another, aiding adaptation and potentially contributing to speciation if these genes lead to reproductive isolation.
- Example: Introgression of genes for pesticide resistance from one insect population to another can lead to ecological specialization and eventual speciation.
Maintaining Species Boundaries:
- Reinforcement: In regions where gene flow occurs between diverging populations, natural selection may strengthen reproductive barriers to maintain species integrity.
- Example: If hybrids have reduced fitness, selection may favor traits that prevent interbreeding, reinforcing speciation.
Creating Genetic Clines:
- Gradual Changes: Gene flow can lead to gradual changes in allele frequencies across geographic gradients, resulting in clinal variation rather than discrete speciation.
- Example: Gradual changes in allele frequencies of a trait across a continent due to continuous gene flow can create a cline without leading to distinct species.
Factors Influencing the Role of Gene Flow in Speciation:
- Population Size: Larger populations are less susceptible to the homogenizing effects of gene flow compared to smaller populations.
- Reproductive Barriers: Prezygotic and postzygotic barriers can limit gene flow, facilitating speciation by reducing interbreeding.
- Environmental Heterogeneity: Diverse environments can create different selective pressures, allowing gene flow to introduce beneficial alleles that promote local adaptation and divergence.
- Frequency of Migration: High rates of migration increase gene flow, making speciation less likely, whereas low rates allow populations to diverge.
Examples Illustrating Gene Flow’s Impact:
African Cichlid Fishes:
- Gene Flow: Hybridization between geographically separated lakes has introduced new genetic variations.
- Result: Facilitated rapid speciation and adaptive radiation, leading to the emergence of numerous distinct cichlid species.
Humans:
- Historical Gene Flow: Gene flow between different human populations has increased genetic diversity.
- Result: Enhanced resilience against diseases and adaptation to diverse environments.
Plants with Wide Dispersal Mechanisms:
- Gene Flow: Wind or animal-mediated seed dispersal introduces new alleles across plant populations.
- Result: Maintains genetic diversity and reduces the likelihood of speciation unless strong selective pressures are present.
Conclusion:
Gene flow plays a critical role in maintaining genetic diversity within populations by introducing new alleles and reducing genetic differences between populations. While it can counteract the processes leading to speciation by maintaining genetic similarity, under certain conditions, gene flow can also facilitate speciation by introducing beneficial alleles that promote adaptation and divergence. Understanding the dynamics of gene flow is essential for comprehending the complex mechanisms driving the evolution and diversification of species.
8. How do biogeographical patterns support the theory of evolution, and what insights do they provide about the distribution of species?
Answer:
Biogeographical Patterns Defined:
Biogeography is the study of the distribution of species and ecosystems across geographic space and through geological time. It examines how species are distributed in relation to the Earth’s physical features, climate, and historical events.
Support for the Theory of Evolution:
Endemic Species:
- Definition: Species that are found exclusively in a specific geographic location.
- Significance: High levels of endemism, especially on islands, suggest that species have evolved in isolation from their mainland relatives.
- Example: The unique species of the Galápagos Islands, such as Darwin’s finches, indicate adaptive radiation from common ancestors adapted to different niches.
Continental Drift and Plate Tectonics:
- Historical Connections: The movement of continents over geological time has influenced the distribution and diversification of species.
- Fossil Evidence: Similar fossils found on now-separated continents support the idea that these landmasses were once connected.
- Example: Fossils of the freshwater reptile Mesosaurus are found in both South America and Africa, indicating that these continents were once part of the supercontinent Gondwana.
Island Biogeography:
- Species Diversity on Islands: Islands often have fewer species than mainland areas but exhibit unique evolutionary paths.
- Adaptive Radiation: Limited resources and isolation promote the diversification of species to fill available ecological niches.
- Example: The Hawaiian honeycreepers have diversified into numerous species with varying beak shapes adapted to different food sources.
Gondwanan and Laurasian Distributions:
- Ancient Supercontinents: The distribution of certain species aligns with the ancient supercontinents Gondwana and Laurasia, suggesting common ancestry before continental breakup.
- Example: Marsupials are primarily found in Australia and South America, reflecting their distribution from the ancient supercontinent Gondwana.
Similar Species in Similar Habitats:
- Convergent Evolution: Similar environmental pressures lead to the evolution of similar traits in unrelated species, reflecting adaptation rather than common ancestry.
- Example: The streamlined bodies of dolphins (mammals) and sharks (fish) are adaptations to aquatic life, showcasing convergent evolution.
Biogeographical Barriers:
- Isolation Factors: Geographic barriers such as mountains, rivers, and oceans limit gene flow between populations, promoting speciation.
- Example: The Andes Mountains act as a barrier, leading to distinct species on either side due to limited gene flow and differing environmental conditions.
Insights Provided by Biogeographical Patterns:
Evolutionary History:
- Historical Events: Biogeographical patterns reveal past events like continental drift, glaciations, and the formation of land bridges that have shaped current species distributions.
- Example: The presence of similar species on North America and Asia suggests past land connections via the Bering land bridge.
Adaptive Radiation:
- Niche Exploitation: Biogeography highlights how isolation and environmental opportunities lead to the rapid diversification of species to fill various ecological roles.
- Example: The diversification of cichlid fishes in African Great Lakes showcases adaptive radiation driven by varying ecological niches.
Speciation Mechanisms:
- Geographical Isolation: Biogeographical patterns support allopatric speciation by showing how physical separation leads to the divergence of species.
- Example: The separation of island populations from mainland populations results in distinct evolutionary trajectories and speciation.
Conservation Biology:
- Biodiversity Hotspots: Identifying regions with high levels of endemism and unique species helps prioritize areas for conservation efforts.
- Example: The Madagascar rainforest is a biodiversity hotspot with numerous endemic species, making it a key focus for conservation.
Understanding Dispersal Mechanisms:
- Species Movement: Biogeography provides insights into how species disperse across landscapes and oceans, influencing their distribution and genetic diversity.
- Example: Birds with long-distance migratory patterns contribute to gene flow between distant populations, affecting genetic structure.
Conclusion:
Biogeographical patterns offer robust support for the theory of evolution by illustrating how species have diversified and adapted to various environments over time. The distribution of species across continents, islands, and different habitats reflects historical processes like continental drift, adaptive radiation, and speciation mechanisms. Studying these patterns enhances our understanding of evolutionary history, species interactions, and the factors driving biodiversity, providing valuable insights for both evolutionary biology and conservation efforts.
9. How do vestigial structures provide evidence for evolution, and can you provide examples from different organisms?
Answer:
Vestigial Structures Defined:
Vestigial structures are anatomical features that have lost most or all of their original function through the course of evolution. These structures are remnants of organs or parts that were functional in ancestral species but have become reduced or obsolete in descendants.
Evidence for Evolution Provided by Vestigial Structures:
- Ancestral Origins: Vestigial structures indicate that an organism has evolved from ancestors with fully functional versions of these structures.
- Evolutionary Modification: The presence of vestigial structures demonstrates how organisms can undergo structural changes over time, adapting to new environments and lifestyles.
- Predictive Power: The existence of vestigial structures allows scientists to make predictions about the evolutionary history of organisms and verify them through comparative anatomy and the fossil record.
Examples of Vestigial Structures in Different Organisms:
Humans:
- Appendix: Once part of the digestive system for fermenting cellulose in ancestral herbivores, the human appendix is now largely redundant, though it may have minor immune functions.
- Wisdom Teeth: Evolved for grinding plant material in ancestors with larger jaws, but often impacted due to reduced jaw size in modern humans.
- Tailbone (Coccyx): The remnant of a lost tail, serving as an anchor point for muscles and ligaments in the pelvic region.
Whales:
- Pelvic Bones: Remnants of hind limbs from their terrestrial ancestors, no longer used for walking but serving as anchor points for reproductive organs.
- Hearing Structures: Vestigial ear bones that no longer play a role in terrestrial hearing but are part of the whale’s auditory system adapted for underwater acoustics.
Birds:
- Wing Claws: Present in some bird species like the hoatzin chicks, vestigial in most adult birds, indicating ancestral climbing abilities.
- Pelvic Bones: Reduced in size and function, reflecting the transition from ground-dwelling to primarily flying lifestyles.
Snakes:
- Hind Limb Remnants: Vestigial pelvic spurs in some snake species like boas and pythons, remnants of their limbed ancestors, used for mating.
- Spurs: Small, claw-like structures near the cloaca, vestigial limbs that no longer serve a locomotor function.
Frogs:
- Tail in Adult Frogs: Some species retain a tadpole-like tail during their larval stage, but it disappears as they metamorphose into adults, leaving behind no functional tail.
Flightless Birds:
- Wings in Ostriches and Emus: Serve other functions such as balance or display, but are incapable of flight.
- Flight Muscles: Reduced in size due to the lack of need for powered flight.
Insects:
- Wings in Some Ant Species: Worker ants in certain species have vestigial wings, remnants from their reproductive or ancestral forms.
- Eyes in Cave-Dwelling Species: Some cave insects have reduced or non-functional eyes, reflecting their adaptation to dark environments where vision is unnecessary.
Significance of Vestigial Structures in Understanding Evolution:
- Demonstrate Ancestral Traits: Highlight features that were functional in ancestors, providing a link between present-day species and their evolutionary history.
- Show Evolutionary Change: Illustrate how species adapt and modify structures over time in response to changing environments and lifestyles.
- Support Common Descent: Provide anatomical evidence that different species share common ancestors, reinforcing the interconnectedness of life through evolution.
Conclusion:
Vestigial structures are powerful evidence for the theory of evolution, showcasing the remnants of ancestral organs that have lost their original functions. These structures across various organisms highlight the dynamic nature of evolution, demonstrating how species adapt, diversify, and sometimes lose traits as they evolve to fit new ecological niches.
10. What is the role of genetic mutations in evolution, and how can they lead to the development of new traits within a population?
Answer:
Genetic Mutations Defined:
Genetic mutations are changes in the DNA sequence of an organism’s genome. They can occur spontaneously during DNA replication or be induced by environmental factors such as radiation, chemicals, or viruses.
Role of Genetic Mutations in Evolution:
Source of Genetic Variation:
- Introducing New Alleles: Mutations create new alleles, contributing to the genetic diversity within a population.
- Allelic Diversity: Increased genetic variation provides the raw material for natural selection to act upon, facilitating adaptation and evolutionary change.
Driving Force for Evolutionary Change:
- Novel Traits: Mutations can result in new or altered traits that may enhance an organism’s fitness or reduce it, depending on the environment.
- Adaptive Evolution: Beneficial mutations increase an organism’s chances of survival and reproduction, spreading advantageous traits through the population.
Facilitating Speciation:
- Genetic Divergence: Accumulation of mutations in isolated populations can lead to genetic divergence, potentially resulting in the formation of new species.
- Reproductive Isolation: Mutations that affect mating behaviors or reproductive compatibility can contribute to reproductive isolation, a key factor in speciation.
Types of Genetic Mutations:
Point Mutations:
- Substitutions: Replacement of one nucleotide with another (e.g., missense, nonsense mutations).
- Impact: Can alter protein function, sometimes leading to new traits or diseases.
Insertions and Deletions (Indels):
- Addition or Removal of Nucleotides: Can cause frameshift mutations, altering the reading frame of genes.
- Impact: Often leads to nonfunctional proteins, but can occasionally create novel protein functions.
Duplication Mutations:
- Replication of Genetic Material: Result in multiple copies of a gene or genomic region.
- Impact: Provides genetic redundancy, allowing one copy to acquire new functions without detrimental effects on the organism.
Inversion and Translocation Mutations:
- Chromosomal Rearrangements: Sections of chromosomes are flipped (inversion) or moved to different locations (translocation).
- Impact: Can disrupt gene function or create new gene combinations, potentially leading to new traits.
Mechanisms by Which Mutations Lead to New Traits:
Gene Expression Changes:
- Regulatory Mutations: Alterations in regulatory regions (promoters, enhancers) can change when, where, and how much a gene is expressed, leading to variations in traits.
- Example: Changes in regulatory genes can result in different flower colors or sizes.
Protein Function Alterations:
- Amino Acid Changes: Mutations can change the amino acid sequence of proteins, affecting their structure and function.
- Example: A single amino acid change in hemoglobin can lead to sickle cell anemia, illustrating how mutations can have significant phenotypic effects.
Gene Duplication and Divergence:
- Functional Redundancy: Duplication allows one gene copy to maintain its original function while the other is free to accumulate mutations that may lead to new functions.
- Example: The evolution of globin genes, where gene duplication led to the development of different hemoglobin types for oxygen transport in various tissues.
Loss of Function Mutations:
- Gene Inactivation: Mutations can render genes nonfunctional, leading to the loss of certain traits.
- Example: Vestigial structures, such as the human appendix, result from mutations that have inactivated their original functions.
Gain of Function Mutations:
- New Functions: Mutations can confer new abilities or enhance existing ones, contributing to adaptive traits.
- Example: The development of antibiotic resistance in bacteria through mutations that alter target sites or increase efflux pump activity.
Impact of Mutations on Population Genetics:
- Allele Frequency Changes: Mutations introduce new alleles, altering allele frequencies within a population over time.
- Evolutionary Potential: Higher mutation rates can increase the evolutionary potential of a population, enabling rapid adaptation to changing environments.
- Genetic Load: Accumulation of deleterious mutations can negatively impact population fitness, but mechanisms like purifying selection help remove harmful alleles.
Conclusion:
Genetic mutations are essential drivers of evolutionary change, introducing the genetic diversity necessary for populations to adapt and evolve. By generating new alleles and altering existing ones, mutations facilitate the development of novel traits, contribute to speciation, and enhance the adaptive potential of organisms. Understanding the role of mutations in evolution is fundamental to comprehending the mechanisms underlying the diversity of life on Earth.
11. How does sexual selection differ from natural selection, and what are some examples of traits that have evolved primarily through sexual selection?
Answer:
Sexual Selection Defined:
Sexual selection is a mode of natural selection where certain traits increase an individual’s chances of successfully mating and reproducing. It arises from the differential reproductive success based on traits that are favored by the opposite sex or by intrasexual competition.
Differences Between Sexual Selection and Natural Selection:
Selection Pressure:
- Natural Selection: Driven by survival-related traits that enhance an organism’s ability to survive in its environment (e.g., camouflage, efficient metabolism).
- Sexual Selection: Driven by traits that enhance an organism’s ability to obtain mates, which may not necessarily improve survival and can sometimes even be detrimental.
Types of Traits Selected:
- Natural Selection: Traits that improve an organism’s fitness by increasing survival and overall reproductive success.
- Sexual Selection: Traits that increase mating success, such as elaborate ornaments, displays, or behaviors, even if they incur a survival cost.
Mechanisms:
- Natural Selection: Involves both interspecific and intraspecific interactions, focusing on traits that confer a survival advantage.
- Sexual Selection: Primarily involves intraspecific interactions, focusing on mate choice and competition for mates.
Types of Sexual Selection:
Intersexual Selection (Mate Choice):
- Definition: Selection where one sex (usually females) chooses mates based on certain desirable traits.
- Outcome: Traits preferred by the selecting sex become more prevalent in the population.
- Example: Female peacocks choosing males with extravagant tail feathers.
Intrasexual Selection (Competition):
- Definition: Selection where members of the same sex (usually males) compete with each other for access to mates.
- Outcome: Traits that enhance competitive abilities become more prevalent.
- Example: Male deer developing large antlers to fight rivals for access to females.
Examples of Traits Evolved Primarily Through Sexual Selection:
Peacock’s Tail:
- Description: Male peacocks possess large, colorful tail feathers used in courtship displays.
- Function: The elaborate tails attract female peahens, signaling the male’s fitness.
- Evolutionary Impact: Despite being cumbersome and energy-intensive to maintain, the tails persist because they increase mating success.
Lion’s Mane:
- Description: Male lions have prominent manes surrounding their heads.
- Function: The mane serves as a display of strength and virility, deterring rivals and attracting females.
- Evolutionary Impact: Males with fuller manes are more likely to secure mates and protect the pride, enhancing reproductive success.
Gorilla’s Chest Pouch:
- Description: Male gorillas have large chest pouches.
- Function: The pouch emphasizes their muscular build, signaling dominance and fitness to females and rival males.
- Evolutionary Impact: Males with larger pouches are more successful in mating and defending their group.
Hummingbird’s Iridescent Plumage:
- Description: Male hummingbirds display bright, iridescent feathers.
- Function: The vibrant colors attract females and indicate the male’s health and genetic quality.
- Evolutionary Impact: Males with more striking plumage have higher mating success, promoting the spread of these traits.
Elephant Seal’s Size:
- Description: Male elephant seals are significantly larger than females.
- Function: Larger size allows males to dominate territories and compete effectively for access to females.
- Evolutionary Impact: Males with greater size and strength secure more breeding opportunities, driving the evolution of gigantism in the species.
Bird-of-Paradise’s Elaborate Displays:
- Description: Male birds-of-paradise perform intricate dances and display vibrant feathers.
- Function: These displays attract females by showcasing the male’s vitality and genetic fitness.
- Evolutionary Impact: The complexity and beauty of the displays are favored, leading to the diversification of species with unique ornamental traits.
Stallion’s Mane and Behavior:
- Description: Stallions exhibit prominent manes and aggressive behaviors during mating seasons.
- Function: The mane and behavior signal dominance and attractiveness to mares.
- Evolutionary Impact: Stallions with more impressive manes and assertive behaviors have higher reproductive success.
Consequences of Sexual Selection:
- Exaggerated Traits: Traits favored by sexual selection can become highly exaggerated, sometimes at the expense of survival.
- Sexual Dimorphism: Significant differences in appearance or behavior between males and females of a species often result from sexual selection.
- Speciation: Divergent sexual preferences can lead to reproductive isolation and eventually speciation.
Conclusion:
Sexual selection is a powerful evolutionary force that shapes the diversity of traits observed in the animal kingdom. By favoring traits that enhance mating success, sexual selection drives the development of elaborate and often costly features, contributing to the rich tapestry of life’s morphological and behavioral diversity.
12. What strategies can be employed in conservation biology to protect and restore ecosystems and their biodiversity?
Answer:
Conservation Biology Defined:
Conservation biology is a multidisciplinary field focused on understanding and mitigating the loss of biodiversity, protecting ecosystems, and ensuring the sustainable management of natural resources. It employs scientific principles to develop strategies aimed at preserving species, habitats, and ecological processes.
Strategies in Conservation Biology:
Protected Areas and Reserves:
- Establishment of Protected Zones: Designating national parks, wildlife reserves, and marine protected areas to safeguard critical habitats and species.
- Impact: Prevent habitat destruction, reduce human disturbances, and provide refuges for endangered species.
- Example: The creation of the Great Barrier Reef Marine Park protects diverse marine life and supports sustainable tourism.
Habitat Restoration and Rehabilitation:
- Ecosystem Restoration: Rehabilitating degraded or destroyed ecosystems to restore their structure, function, and biodiversity.
- Techniques: Reforestation, wetland restoration, removal of invasive species, and reintroduction of native species.
- Example: Restoring wetlands in Louisiana to support migratory birds and improve water quality.
Species Reintroduction and Recovery Programs:
- Reintroduction: Bringing back species that have been extirpated from certain areas to restore ecological balance.
- Recovery Programs: Targeted efforts to increase populations of endangered or threatened species.
- Example: The reintroduction of gray wolves to Yellowstone National Park has restored trophic cascades and ecosystem health.
Invasive Species Management:
- Control and Eradication: Implementing measures to control or eliminate invasive species that threaten native biodiversity.
- Preventative Actions: Biosecurity measures to prevent the introduction and spread of invasive species.
- Example: Removing invasive plants like kudzu to allow native vegetation to recover and support local wildlife.
Ex Situ Conservation:
- Captive Breeding: Breeding endangered species in controlled environments like zoos and botanical gardens.
- Seed Banks: Preserving seeds of plant species for future restoration and reintroduction.
- Genetic Repositories: Maintaining genetic diversity through DNA banks and biobanks.
- Example: The Svalbard Global Seed Vault stores seeds from around the world to safeguard against catastrophic loss.
Sustainable Resource Management:
- Fisheries Management: Implementing quotas, marine protected areas, and sustainable fishing practices to prevent overfishing.
- Forestry Practices: Promoting selective logging, reforestation, and sustainable timber harvesting to maintain forest ecosystems.
- Agricultural Sustainability: Encouraging practices like crop rotation, organic farming, and integrated pest management to preserve soil health and biodiversity.
- Example: The use of sustainable forestry certifications (e.g., FSC) ensures that timber is harvested responsibly, protecting forest ecosystems.
Climate Change Mitigation and Adaptation:
- Reducing Emissions: Implementing policies and practices to lower greenhouse gas emissions, thereby mitigating climate change impacts.
- Enhancing Resilience: Developing strategies to help ecosystems and species adapt to changing climatic conditions.
- Example: Coastal mangrove restoration enhances carbon sequestration, provides storm protection, and supports marine biodiversity.
Community-Based Conservation:
- Engaging Local Communities: Involving indigenous peoples and local stakeholders in conservation efforts ensures that strategies are culturally appropriate and gain community support.
- Empowering Communities: Providing education, resources, and incentives for sustainable practices.
- Example: Community-managed forests in Nepal balance timber production with conservation, benefiting both people and biodiversity.
Legal and Policy Frameworks:
- Enforcing Laws: Implementing and enforcing environmental protection laws and regulations to safeguard habitats and species.
- International Agreements: Participating in global treaties like the Convention on Biological Diversity (CBD) to promote international cooperation.
- Example: The Endangered Species Act (ESA) in the United States provides legal protection for threatened and endangered species.
Biodiversity Monitoring and Research:
- Data Collection: Conducting surveys, tracking populations, and monitoring ecosystem health to inform conservation strategies.
- Scientific Research: Studying ecological processes, species interactions, and the impacts of human activities to develop evidence-based conservation approaches.
- Example: Long-term monitoring of bird populations helps detect declines early, allowing for timely conservation interventions.
Restoration of Ecosystem Functions:
- Functional Restoration: Reestablishing key ecological processes like pollination, nutrient cycling, and natural fire regimes to enhance ecosystem health.
- Example: Reintroducing fire in fire-dependent ecosystems like prairies to maintain plant diversity and prevent woody plant encroachment.
Public Awareness and Education:
- Raising Awareness: Educating the public about the importance of biodiversity and the threats it faces encourages support for conservation initiatives.
- Promoting Stewardship: Fostering a sense of responsibility and encouraging individuals to participate in conservation activities.
- Example: Campaigns like Earth Hour inspire global participation in environmental protection efforts.
Challenges in Conservation Biology:
- Funding and Resources:
- Limited Funding: Adequate financial resources are often scarce, hindering the implementation of comprehensive conservation programs.
- Political and Economic Barriers:
- Policy Inconsistencies: Political instability, lack of enforcement, and conflicting economic interests can impede conservation efforts.
- Climate Change:
- Dynamic Conditions: Rapid environmental changes make it difficult to predict and respond to conservation needs effectively.
- Invasive Species:
- Persistent Threats: Controlling invasive species requires sustained efforts and can be resource-intensive.
- Human-Wildlife Conflicts:
- Competing Interests: Balancing human development and wildlife conservation can lead to conflicts and resistance from local communities.
Conclusion:
Conservation biology employs a multifaceted approach to protect and restore ecosystems and their biodiversity. Strategies range from establishing protected areas and restoring habitats to managing invasive species and engaging local communities. Overcoming challenges such as limited funding, political barriers, and climate change is essential for the success of conservation efforts. By integrating scientific research, sustainable practices, and community involvement, conservation biology aims to preserve the intricate web of life that sustains ecosystems and, ultimately, human well-being.
Bonus Question: How do biogeographical patterns support the theory of evolution, and what insights do they provide about the distribution of species?
Answer:
Biogeographical Patterns Defined:
Biogeography is the study of the distribution of species and ecosystems across geographic space and through geological time. It examines how species are distributed in relation to the Earth’s physical features, climate, and historical events.
Support for the Theory of Evolution:
Endemic Species:
- Definition: Species that are found exclusively in a specific geographic location.
- Significance: High levels of endemism, especially on islands, suggest that species have evolved in isolation from their mainland relatives.
- Example: The unique species of the Galápagos Islands, such as Darwin’s finches, indicate adaptive radiation from common ancestors adapted to different niches.
Continental Drift and Plate Tectonics:
- Historical Connections: The movement of continents over geological time has influenced the distribution and diversification of species.
- Fossil Evidence: Similar fossils found on now-separated continents support the idea that these landmasses were once connected.
- Example: Fossils of the freshwater reptile Mesosaurus are found in both South America and Africa, indicating that these continents were once part of the supercontinent Gondwana.
Island Biogeography:
- Species Diversity on Islands: Islands often have fewer species than mainland areas but exhibit unique evolutionary paths.
- Adaptive Radiation: Limited resources and isolation promote the diversification of species to fill available ecological niches.
- Example: The Hawaiian honeycreepers have diversified into numerous species with varying beak shapes adapted to different food sources.
Gondwanan and Laurasian Distributions:
- Ancient Supercontinents: The distribution of certain species aligns with the ancient supercontinents Gondwana and Laurasia, suggesting common ancestry before continental breakup.
- Example: Marsupials are primarily found in Australia and South America, reflecting their distribution from the ancient supercontinent Gondwana.
Similar Species in Similar Habitats:
- Convergent Evolution: Similar environmental pressures lead to the evolution of similar traits in unrelated species, reflecting adaptation rather than common ancestry.
- Example: The streamlined bodies of dolphins (mammals) and sharks (fish) are adaptations to aquatic life, showcasing convergent evolution.
Biogeographical Barriers:
- Isolation Factors: Geographic barriers such as mountains, rivers, and oceans limit gene flow between populations, promoting speciation.
- Example: The Andes Mountains act as a barrier, leading to distinct species on either side due to limited gene flow and differing environmental conditions.
Insights Provided by Biogeographical Patterns:
Evolutionary History:
- Historical Events: Biogeographical patterns reveal past events like continental drift, glaciations, and the formation of land bridges that have shaped current species distributions.
- Example: The presence of similar species on North America and Asia suggests past land connections via the Bering land bridge.
Adaptive Radiation:
- Niche Exploitation: Biogeography highlights how isolation and environmental opportunities lead to the rapid diversification of species to fill various ecological roles.
- Example: The diversification of cichlid fishes in African Great Lakes showcases adaptive radiation driven by varying ecological niches.
Speciation Mechanisms:
- Geographical Isolation: Biogeographical patterns support allopatric speciation by showing how physical separation leads to the divergence of species.
- Example: The separation of island populations from mainland populations results in distinct evolutionary trajectories and speciation.
Conservation Biology:
- Biodiversity Hotspots: Identifying regions with high levels of endemism and unique species helps prioritize areas for conservation efforts.
- Example: The Madagascar rainforest is a biodiversity hotspot with numerous endemic species, making it a key focus for conservation.
Understanding Dispersal Mechanisms:
- Species Movement: Biogeography provides insights into how species disperse across landscapes and oceans, influencing their distribution and genetic diversity.
- Example: Birds with long-distance migratory patterns contribute to gene flow between distant populations, affecting genetic structure.
Conclusion:
Biogeographical patterns offer robust support for the theory of evolution by illustrating how species have diversified and adapted to various environments over time. The distribution of species across continents, islands, and different habitats reflects historical processes like continental drift, adaptive radiation, and speciation mechanisms. Studying these patterns enhances our understanding of evolutionary history, species interactions, and the factors driving biodiversity, providing valuable insights for both evolutionary biology and conservation efforts.