Cell Physiology and Biochemistry
Cell physiology explores the vital functions and biochemical processes that enable cells to sustain life, communicate, and adapt to their environment. As a subfield of science, it forms a crucial part of the study of biology and plays a foundational role in the broader discipline of cell biology. Students of cell physiology investigate the dynamic processes that maintain homeostasis, regulate metabolism, and support survival. These include signal transduction pathways detailed in cell communication, and the regulated series of events known as the cell cycle.
Cellular behavior is also shaped during cell development, when physiological functions are assigned to different cell types. The structural components that support and enable cellular functions are explored in cell structure, while broader ecological relationships are addressed in ecology. The evolutionary significance of physiological adaptations is covered in evolutionary biology. These physiological mechanisms are often encoded in the genetic instructions inherited across generations, which are studied in genetics and genomics.
To understand how cellular functions are inherited and regulated, students delve into Mendelian genetics and molecular genetics. These topics help explain how genes control enzyme activity, transport mechanisms, and energy conversion in the cell. At the core of this is the structure and interaction of DNA and RNA, along with the processes of gene expression and protein synthesis. When these processes malfunction, it can lead to genetic mutation and altered cell behavior, which are crucial considerations in medical and research contexts.
The study of molecular basis of inheritance provides a framework for understanding how physiological traits are passed down and expressed. Such insights are complemented by investigations into molecular evolution and the use of molecular techniques in research. Applications in biotechnology and healthcare—such as those in applications of genetics in medicines and DNA technology—highlight how cell physiology knowledge is translated into real-world innovations.
On a population level, physiological traits are explored through population genetics and quantitative genetics, offering a broader lens on how cellular functions impact organisms and species. Through this interconnected web of knowledge, cell physiology empowers students to grasp life at its most fundamental level, forming a basis for future exploration in biological science and biomedical fields.
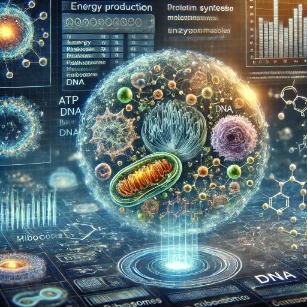
Table of Contents
Overview of Cellular Metabolic Pathways
Metabolism encompasses the entire set of life-sustaining chemical transformations occurring within the cells of organisms. These reactions provide the energy and molecular building blocks necessary for growth, reproduction, and homeostasis. Metabolic pathways are typically categorized into two broad groups:
- Catabolic pathways: These involve the breakdown of complex molecules like carbohydrates, lipids, and proteins into simpler compounds, releasing energy in the form of ATP. They are essential for supplying the cellular machinery with energy to perform functions like active transport, mechanical work, and biosynthesis.
- Anabolic pathways: These utilize energy to build complex biomolecules from simpler precursors. Anabolism supports cell growth, repair, and maintenance by synthesizing macromolecules like nucleic acids, proteins, and phospholipids.
Glycolysis
- Definition: Glycolysis is a universal metabolic pathway that breaks down glucose (C₆H₁₂O₆) into two molecules of pyruvate, generating a net gain of ATP and NADH. It is the first step of cellular respiration and occurs in nearly all organisms.
- Location: Occurs in the cytoplasm and does not require oxygen, making it especially crucial for anaerobic organisms and hypoxic cellular conditions.
- Importance:
- Provides rapid ATP production, especially in tissues with high energy demand like muscles.
- Supplies intermediates for various biosynthetic pathways including amino acid and nucleotide synthesis.
- Feeds into both aerobic (Krebs cycle and ETC) and anaerobic (fermentation) metabolic fates depending on oxygen availability.
- Net Reaction:
The Krebs Cycle (Citric Acid Cycle)
- Definition: The Krebs cycle is a central metabolic pathway that completes the oxidation of glucose-derived carbon skeletons. It processes acetyl-CoA into CO₂ and harnesses high-energy electrons in the form of NADH and FADH₂.
- Location: Takes place in the mitochondrial matrix and is tightly linked to aerobic respiration.
- Role:
- Generates key electron carriers (NADH and FADH₂) that feed into the electron transport chain.
- Produces GTP/ATP directly via substrate-level phosphorylation.
- Provides biosynthetic precursors for amino acids, nucleotides, and heme groups.
- Key Products per cycle (per acetyl-CoA):
- 3 NADH, 1 FADH₂, 1 ATP (or GTP), and 2 CO₂.
Oxidative Phosphorylation
- Definition: This process synthesizes ATP by using the energy derived from the transfer of electrons through the electron transport chain (ETC) to molecular oxygen, coupled with proton pumping and chemiosmosis.
- Location: Occurs across the inner mitochondrial membrane, which houses the ETC protein complexes and ATP synthase.
- Steps:
- Electron Transport Chain: Electrons from NADH and FADH₂ are shuttled through protein complexes I-IV. The redox reactions release energy at each step, enabling proton pumping into the intermembrane space.
- Proton Gradient Formation: A steep electrochemical gradient (proton motive force) is established as H⁺ ions accumulate in the intermembrane space.
- ATP Synthesis: As protons flow back into the matrix via ATP synthase, mechanical energy is converted into chemical energy, forming ATP from ADP and Pi.
- Importance: Oxidative phosphorylation produces about 28-34 molecules of ATP per glucose, making it the most efficient energy-producing process in eukaryotic cells.
- Net Reaction:
Altogether, these metabolic pathways—glycolysis, the Krebs cycle, and oxidative phosphorylation—form the backbone of cellular respiration. Their integration allows cells to dynamically adjust their energy production according to oxygen availability, energy demand, and nutrient supply. Additionally, intermediates from these pathways feed into biosynthetic routes for nucleotides, amino acids, lipids, and other essential compounds, highlighting their central role in cellular metabolism.
Advancements in metabolomics, mitochondrial biology, and systems biochemistry have deepened our understanding of these processes, enabling medical breakthroughs in diagnosing and treating metabolic disorders. A detailed resource on these topics is available from Khan Academy’s guide on cellular respiration, which offers diagrams and interactive explanations for all major steps.
Protein Synthesis and Enzyme Activity
Protein Synthesis
Protein synthesis is a fundamental cellular process through which the genetic code stored in DNA is translated into functional proteins—molecules that perform structural, regulatory, catalytic, and signaling functions. This process is essential for cell growth, maintenance, and response to environmental stimuli. Protein synthesis involves a tightly regulated, two-step sequence:
- Transcription: This step occurs in the cell nucleus where a specific segment of DNA is copied into messenger RNA (mRNA) by the enzyme RNA polymerase. The resulting mRNA strand carries the genetic information encoded in DNA to the ribosomes, acting as a molecular blueprint for the protein.
- Translation: Once in the cytoplasm, the mRNA is read by ribosomes—a complex composed of ribosomal RNA and protein. Transfer RNA (tRNA) molecules bring the appropriate amino acids in sequence according to the codons on the mRNA. These amino acids are linked together via peptide bonds to form a polypeptide chain that folds into a functional protein.
This central dogma of molecular biology—DNA to RNA to protein—ensures the flow of genetic information and allows cells to adapt and respond through protein expression.
Proteins serve as:
- Structural components: Proteins like actin, tubulin, and keratin form the cytoskeleton, giving shape and mechanical strength to cells and tissues. In connective tissues, collagen fibers are critical for integrity and flexibility.
- Enzymes: These specialized proteins catalyze thousands of biochemical reactions required for life. For instance, DNA polymerase is crucial for DNA replication, while amylase breaks down starch into sugars in digestion.
- Transporters and Receptors: Membrane proteins like GLUT transport glucose across membranes, while receptor proteins such as insulin receptors initiate cellular responses to external signals.
- Hormones: Protein-based hormones such as insulin, growth hormone, and oxytocin regulate a wide range of physiological processes including metabolism, development, and social behavior.
Enzyme Activity
Enzymes are highly specific biological catalysts that enable and accelerate chemical reactions by lowering the activation energy required. Without enzymes, most biochemical reactions would proceed too slowly to sustain life. Enzymes exhibit remarkable specificity for their substrates and operate under mild physiological conditions, making them ideal for biological systems.
- Mechanism:
- Enzymes function by binding to their substrate(s) at the active site, a region with a specific three-dimensional shape and chemical environment.
- This binding forms a transient enzyme-substrate complex that stabilizes the reaction’s transition state, greatly enhancing the reaction rate.
- Once the reaction occurs, the product is released and the enzyme is regenerated, ready to catalyze another cycle.
- Factors Influencing Enzyme Activity:
- Temperature: Enzymes have an optimal temperature at which their activity is highest. For most human enzymes, this is around 37°C. Excess heat can denature the enzyme, while low temperatures reduce kinetic energy and reaction rates.
- pH: Each enzyme functions optimally within a specific pH range. For example, pepsin in the stomach works best in acidic conditions (pH ~2), whereas trypsin in the small intestine requires a neutral to slightly basic environment.
- Inhibitors: Molecules that reduce enzyme activity fall into two major categories:
- Competitive inhibitors bind to the active site, preventing substrate access (e.g., methotrexate inhibits dihydrofolate reductase).
- Non-competitive inhibitors bind elsewhere on the enzyme, altering its shape and function without competing directly for the active site.
- Cofactors and Coenzymes: Many enzymes require additional molecules to function. Metal ions like Mg²⁺ or organic cofactors like NAD⁺ participate in catalytic activity or substrate binding.
Enzymes are not only central to cellular metabolism but also play indispensable roles in biotechnology and medicine. They are used in diagnostics, drug design, industrial catalysis, and food processing. A foundational understanding of enzymes is essential for developing therapies for metabolic diseases, infections, and cancer.
To explore protein synthesis and enzyme mechanisms with interactive animations and detailed pathways, visit this educational guide on the National Center for Biotechnology Information (NCBI).
Signal Transduction Pathways
Signal transduction is a vital process by which cells perceive and appropriately respond to external cues, ranging from hormones and growth factors to environmental stressors. These signaling mechanisms enable multicellular organisms to coordinate complex behaviors such as growth, immune defense, development, and homeostasis. At its core, signal transduction converts an extracellular signal into a specific cellular action, often through a series of finely tuned molecular events.
Key Steps in Signal Transduction
- Reception: The process begins when signaling molecules, or ligands, such as hormones, neurotransmitters, or cytokines, bind to their corresponding receptors. These receptors can be located:
- On the cell surface (e.g., G-protein coupled receptors, tyrosine kinase receptors) for water-soluble ligands.
- Inside the cell (e.g., steroid hormone receptors) for lipophilic ligands that can cross the membrane.
- Transduction: The initial signal is propagated and amplified through a sequence of intracellular events involving:
- Second messengers such as cyclic AMP (cAMP), calcium ions (Ca²⁺), and inositol trisphosphate (IP₃), which diffuse rapidly to relay the message.
- Protein kinases and phosphatases, which modify target proteins by phosphorylation or dephosphorylation, altering their activity and interactions.
- Response: The final outcome of signal transduction can vary widely depending on the signal type and cell context:
- Activation or repression of gene expression through transcription factors such as CREB or NF-κB.
- Modulation of enzyme activity, leading to changes in metabolism or ion transport.
- Initiation of processes such as cell division, differentiation, apoptosis, or immune responses.
Major Examples of Signal Transduction Pathways
- G-Protein Coupled Receptor (GPCR) Pathways: These are the most diverse class of signaling pathways in eukaryotes. Upon ligand binding, the receptor activates a G-protein, which in turn modulates effectors such as adenylyl cyclase or phospholipase C. GPCRs regulate a broad range of physiological functions including:
- Metabolism: Regulation of glucose and lipid metabolism via hormones like glucagon and adrenaline.
- Sensory perception: Vision (via rhodopsin in the retina), taste, and smell rely on GPCR signaling.
- Immune response: GPCRs mediate chemokine signaling that guides immune cells to infection sites.
- MAPK (Mitogen-Activated Protein Kinase) Pathway: A critical kinase cascade that relays signals from the cell membrane to the nucleus. It is typically activated by growth factors or stress stimuli and includes ERK, JNK, and p38 MAPK branches. Its major roles include:
- Cell growth and proliferation: Frequently activated in response to epidermal growth factor (EGF).
- Cell differentiation: Especially in stem cells and during development.
- Apoptosis and stress response: Triggered by UV radiation, inflammatory cytokines, or oxidative stress.
- JAK-STAT Pathway: Used by many cytokines and growth factors. The receptor-associated Janus kinases (JAKs) phosphorylate STAT transcription factors, which dimerize and translocate to the nucleus to modulate gene expression. This pathway plays a crucial role in hematopoiesis, immune function, and inflammation.
- PI3K-Akt Pathway: Activated by receptor tyrosine kinases (e.g., insulin receptor), this pathway regulates metabolism, cell survival, and angiogenesis. Aberrations in PI3K-Akt signaling are linked to cancer, insulin resistance, and cardiovascular disease.
Clinical Relevance and Therapeutic Targeting
Dysregulation of signal transduction pathways is a hallmark of many diseases. For example, overactivation of the MAPK or PI3K-Akt pathways can lead to uncontrolled cell proliferation and cancer. Pharmacological agents that target specific components of these pathways—such as kinase inhibitors or monoclonal antibodies—are used in the treatment of cancers, autoimmune diseases, and metabolic disorders. This article on NCBI offers a comprehensive review of therapeutic strategies involving signal transduction modulators.
Additionally, research into synthetic biology and cell-based therapies is exploring how engineered signal transduction systems can be used to design smart drugs or programmable immune responses, further underscoring the significance of this field.
Cellular Energy Production and Management
All living cells require a continuous and reliable supply of energy to maintain life processes, including biosynthesis of macromolecules, active transport of substances, muscle contraction, signal transmission, and cellular replication. The main energy carrier that enables these activities is adenosine triphosphate (ATP), a high-energy molecule capable of storing and releasing energy in a form readily usable by enzymes and other cellular machinery. Understanding the mechanisms of energy production and the strategies cells use to manage their energy budget is central to cell biology, physiology, and bioengineering.
ATP Production Mechanisms
Cells synthesize ATP through multiple biochemical pathways, each adapted to specific cellular environments and resource availability:
- Substrate-Level Phosphorylation: This is the direct transfer of a phosphate group from a high-energy substrate molecule to ADP, forming ATP. It occurs during:
- Glycolysis in the cytoplasm: For example, phosphoenolpyruvate (PEP) donates a phosphate to ADP via the enzyme pyruvate kinase.
- Krebs cycle in the mitochondrial matrix: Specifically, during the conversion of succinyl-CoA to succinate.
- Oxidative Phosphorylation: This is the primary ATP-producing process in aerobic organisms. It occurs in the inner mitochondrial membrane where:
- Electrons from NADH and FADH2 are transferred through the electron transport chain (ETC).
- Energy from this transfer pumps protons (H⁺) into the intermembrane space, creating an electrochemical gradient.
- ATP synthase utilizes this gradient to catalyze ATP formation from ADP and inorganic phosphate.
- Photophosphorylation: Occurring in chloroplasts of plant cells and cyanobacteria, this process uses solar energy to drive the formation of ATP during the light-dependent reactions of photosynthesis.
- Photon absorption by chlorophyll excites electrons that travel through the photosynthetic electron transport chain.
- This generates a proton gradient across the thylakoid membrane, similar to oxidative phosphorylation.
- ATP synthase then produces ATP for use in the Calvin cycle to fix carbon dioxide into glucose.
Energy Storage and Regulation
Because energy demand fluctuates throughout a cell’s life cycle and environmental conditions, cells have evolved strategies to manage energy generation and store excess fuel for future use:
- Allosteric Regulation: Enzymes involved in glycolysis and the Krebs cycle are regulated by molecules such as ATP, AMP, and citrate to match ATP production with demand.
- Hormonal Control: In animals, hormones like insulin and glucagon control glucose uptake and metabolism through second messenger systems.
- Metabolic Flexibility: During intense activity (e.g., exercise), muscle cells switch from oxidative phosphorylation to anaerobic glycolysis for faster ATP production, albeit less efficiently.
Energy Storage Compounds
- Glycogen: In animals, especially liver and muscle cells, excess glucose is stored as glycogen—a highly branched polysaccharide rapidly mobilized when glucose is scarce.
- Starch: In plants, glucose is stored as starch granules in plastids, serving as an energy reserve during periods of darkness or dormancy.
- Lipids: Triglycerides store more energy per gram than carbohydrates and are utilized in energy-demanding conditions like fasting or migration.
Energy Homeostasis in Specialized Cells
Different cell types tailor their energy strategies to suit functional roles:
- Neurons are highly dependent on continuous glucose metabolism due to their high energy demands and limited energy storage.
- Cardiac muscle cells primarily rely on fatty acid oxidation for ATP, ensuring long-term endurance performance.
- Adipocytes specialize in long-term energy storage in the form of lipids and can release fatty acids through lipolysis when energy is needed elsewhere in the body.
Relevance to Health and Disease
Dysfunctions in energy production pathways are central to a range of diseases. Mitochondrial disorders can impair ATP synthesis and lead to neurodegeneration, muscular dystrophy, or metabolic acidosis. In cancer cells, the Warburg effect describes a shift toward glycolysis even in the presence of oxygen, providing a growth advantage but also highlighting potential therapeutic targets. Strategies to manipulate cellular energy—such as ketogenic diets, mitochondrial therapeutics, and exercise regimens—are being explored for treating metabolic and neurodegenerative diseases. Learn more about cellular bioenergetics and metabolic adaptation in complex diseases from Frontiers in Cell and Developmental Biology.
Importance of Cell Physiology and Biochemistry
Cell physiology and biochemistry are foundational disciplines in life sciences that explore the molecular mechanisms and physical principles underlying cellular function. These fields help us understand how cells convert energy, synthesize macromolecules, respond to stimuli, and maintain homeostasis. Insights gained from studying cellular processes inform our understanding of health, disease, and innovation in biotechnology and medicine. The dynamic interactions between enzymes, organelles, signaling pathways, and structural proteins determine the fate and function of every cell in the body, making cellular physiology and biochemistry indispensable in research and clinical practice.
Medical Applications
- Understanding cellular processes enables researchers and physicians to identify the root causes of diseases and design targeted interventions. For example, the regulation of blood glucose levels through insulin signaling pathways is central to the management of type 1 and type 2 diabetes. Disruptions in mitochondrial oxidative phosphorylation lead to a range of mitochondrial disorders, often affecting organs with high energy demands such as the brain, muscles, and heart.
- Drug discovery frequently relies on cellular models to test compounds that modulate biochemical pathways. In cancer therapy, for instance, drugs like kinase inhibitors specifically block aberrant signaling cascades that drive uncontrolled cell division. Similarly, therapies targeting enzymes such as reverse transcriptase have been pivotal in managing HIV/AIDS.
- Personalized medicine uses biochemical and physiological markers from patient cells to tailor treatments, improving outcomes and minimizing side effects. Biomarkers such as HER2 in breast cancer or BCR-ABL in chronic myeloid leukemia have led to more precise and effective treatments.
Biotechnology
- The knowledge of cellular biochemistry is essential for engineering microbes, plant cells, or mammalian cells to produce valuable substances in industrial biotechnology. For instance, optimizing fermentation conditions and metabolic pathways allows for increased yields of biofuels, vitamins, vaccines, and recombinant pharmaceuticals like insulin and monoclonal antibodies.
- In synthetic biology, metabolic engineering involves redesigning cellular pathways to create organisms with new capabilities, such as bacteria that can degrade plastic or yeast strains that synthesize novel antibiotics.
- Advanced biotechnologies like CRISPR-Cas9 rely on deep understanding of nucleic acid biochemistry to perform gene editing. These tools have opened possibilities in gene therapy, agriculture, and even environmental remediation.
Disease Mechanisms
- Diseases often originate from biochemical dysfunctions at the cellular level. For example, mutations in signaling proteins like Ras or PI3K lead to hyperactive proliferation in cancer, while impairments in protein clearance pathways can cause accumulation of toxic aggregates, leading to neurodegenerative diseases such as Alzheimer’s and Parkinson’s.
- Errors in cellular transport, ion channel function, or enzyme catalysis can result in genetic disorders like cystic fibrosis, phenylketonuria, or Tay-Sachs disease. Understanding the underlying biochemical disruptions enables the design of enzyme replacement therapies or gene therapies.
- Inflammation and immune responses also involve complex signaling pathways. Autoimmune diseases can arise from misregulated immune cell activation, while chronic inflammation is a contributing factor in cardiovascular diseases, cancer, and metabolic syndrome.
- Cutting-edge research in cell biology and biochemistry continues to uncover how the malfunction of specific molecular players contributes to human pathologies, paving the way for future diagnostics and treatments.
In summary, the importance of cell physiology and biochemistry cannot be overstated. These disciplines connect molecular science with clinical and industrial applications, driving progress across medicine, biotechnology, pharmacology, and public health.
Why Study Cell Physiology
Understanding Cellular Processes
Cell physiology focuses on the functions and activities that occur within a cell. It helps students understand how nutrients are transported, how energy is generated, and how waste is removed. This foundational knowledge is essential for explaining how cells survive and interact. Grasping these processes is vital for fields such as medicine, pharmacology, and biotechnology.
Linking Structure and Function
Studying cell physiology highlights how cellular structures are optimized for specific functions. For example, mitochondria are adapted to produce energy efficiently through cellular respiration. Understanding this structure-function relationship is key in diagnosing and treating cellular disorders. It enables students to connect theoretical biology with practical medical applications.
Relevance to Human Health
Many diseases originate from physiological malfunctions at the cellular level. By studying these dysfunctions, students can comprehend how illnesses develop and how treatments work. This knowledge forms the basis for careers in clinical research and diagnostics. A deep understanding of cell physiology enhances the ability to innovate in healthcare.
Tools for Research and Innovation
Cell physiology relies on advanced tools such as electrophysiology, imaging, and biochemical assays. Mastering these tools prepares students for research in academic and industrial labs. These techniques allow scientists to investigate how cells respond to different stimuli and drugs. Proficiency in such methods is valuable for future biomedical scientists and engineers.
Preparation for Advanced Biological Studies
A solid grasp of cell physiology is crucial for progressing in molecular biology, biochemistry, and systems biology. It builds the analytical skills needed to study complex life systems. Students learn to interpret experimental data and develop hypotheses. This sets the foundation for academic and professional success in life sciences.
Cell Physiology and Biochemistry: Conclusion
Cell physiology and biochemistry form the cornerstone of modern biological understanding, offering deep insights into the molecular and cellular mechanisms that govern life. These fields bridge the gap between structure and function, connecting genes, proteins, organelles, and systems into a cohesive picture of how living organisms operate. They not only explain how individual cells work but also illuminate how groups of cells coordinate to form tissues, how organs maintain functionality, and how systems respond to changes in internal and external environments.
At the heart of this exploration lies the cell’s remarkable ability to generate and manage energy. Biochemical pathways such as glycolysis, the citric acid cycle, and oxidative phosphorylation underscore the elegance of cellular energetics, converting nutrients into adenosine triphosphate (ATP), the energy currency of life. Without this energy, essential activities like active transport, signal transduction, and cell division would grind to a halt. The regulation of these energy-yielding reactions highlights how tightly controlled and interconnected cellular processes must be to preserve homeostasis.
Protein synthesis and enzyme activity further exemplify the sophistication of the cell. From the transcription of genetic information in the nucleus to the translation and folding of functional proteins in the cytoplasm and endoplasmic reticulum, the journey from gene to protein is complex yet highly orchestrated. Enzymes, the molecular machines of the cell, accelerate thousands of reactions every second, enabling life to proceed at a sustainable pace. Understanding enzyme kinetics and regulation has led to breakthroughs in pharmacology and clinical diagnostics.
Signal transduction pathways provide a communication network that allows cells to sense, interpret, and respond to their environment. Whether it’s the reception of a hormone, a neurotransmitter, or a growth factor, the cascade of intracellular signals that follows ensures the appropriate physiological response. Disruptions in these signaling mechanisms are often at the root of diseases such as cancer, autoimmune conditions, and metabolic disorders, making them key targets for therapeutic intervention.
The implications of research in cell physiology and biochemistry are vast. In medicine, the understanding of cellular processes has led to the development of life-saving treatments, including enzyme replacement therapies, monoclonal antibodies, and precision gene editing technologies. In agriculture, it has improved crop yields and resistance through metabolic engineering. In industrial biotechnology, engineered microbes now produce everything from insulin to biodegradable plastics.
Moreover, studying these processes enhances our ability to model diseases in vitro, enabling scientists to explore treatments in controlled environments before moving to clinical trials. It also informs the design of artificial organs, lab-grown tissues, and even biosensors for environmental monitoring. As researchers continue to dissect these fundamental mechanisms, new horizons emerge in synthetic biology, nanomedicine, and systems biology.
Ultimately, the study of cell physiology and biochemistry is not merely academic—it is a gateway to understanding life at its most intricate and practical level. It empowers us to diagnose and treat illness, engineer sustainable solutions, and push the boundaries of what is biologically possible. For anyone passionate about science, medicine, or innovation, a firm grounding in these areas provides an essential foundation.
To explore current breakthroughs and foundational discoveries in these fields, consider reviewing research curated by journals such as Cell Metabolism, which highlights global advances in cellular energy balance, nutrient sensing, and metabolic disease.
Cell Physiology and Biochemistry: Review Questions and Answers
Question 1:
What are the primary functions of the mitochondria in eukaryotic cells?
Answer:
Mitochondria are often referred to as the “powerhouses” of the cell due to their critical role in energy production. Their primary functions include:
ATP Production: Mitochondria generate adenosine triphosphate (ATP) through oxidative phosphorylation, which occurs in the inner mitochondrial membrane. This process involves the electron transport chain and chemiosmosis, producing the majority of the cell’s ATP.
Citric Acid Cycle (Krebs Cycle): Mitochondria are the site of the citric acid cycle, where acetyl-CoA is oxidized to produce electron carriers (NADH and FADH₂) that feed into the electron transport chain.
Regulation of Cellular Metabolism: Mitochondria play a role in regulating metabolic pathways by controlling the availability of substrates and intermediates for various biochemical reactions.
Apoptosis: Mitochondria release pro-apoptotic factors like cytochrome c, which are essential for the initiation of programmed cell death.
Calcium Homeostasis: Mitochondria help regulate intracellular calcium levels, which are crucial for various cellular signaling pathways.
Heat Production: In brown adipose tissue, mitochondria generate heat through a process called non-shivering thermogenesis, mediated by uncoupling proteins.
Overall, mitochondria are essential for energy production, metabolic regulation, and maintaining cellular homeostasis.
Question 2:
Describe the role of the endoplasmic reticulum in protein synthesis and processing.
Answer:
The endoplasmic reticulum (ER) is a crucial organelle involved in the synthesis, folding, modification, and transport of proteins. It exists in two forms: rough ER (RER) and smooth ER (SER), each with distinct functions related to protein physiology.
Rough Endoplasmic Reticulum (RER):
- Protein Synthesis: The RER is studded with ribosomes, which are the sites of protein synthesis. These ribosomes translate mRNA into polypeptide chains destined for secretion, incorporation into the plasma membrane, or use within lysosomes.
- Protein Folding and Quality Control: As proteins are synthesized, they enter the lumen of the RER, where molecular chaperones assist in proper folding. The RER also ensures quality control by identifying and degrading misfolded proteins through the ER-associated degradation (ERAD) pathway.
- Post-Translational Modifications: Proteins undergo various modifications in the RER, such as glycosylation (addition of carbohydrate groups), which are essential for their stability, functionality, and targeting.
Smooth Endoplasmic Reticulum (SER):
- Lipid Synthesis: The SER is involved in the synthesis of lipids, including phospholipids and cholesterol, which are vital for membrane formation and maintenance.
- Detoxification: In liver cells, the SER contains enzymes that detoxify drugs and harmful substances by chemically modifying them to make them more water-soluble for excretion.
- Calcium Storage and Release: The SER serves as a storage site for calcium ions, which are released into the cytoplasm to participate in various signaling pathways and cellular processes.
Overall, the endoplasmic reticulum is essential for the efficient production, modification, and distribution of proteins, as well as for lipid metabolism and detoxification processes within the cell.
Question 3:
How does the plasma membrane maintain cellular homeostasis?
Answer:
The plasma membrane is a selectively permeable barrier that separates the internal environment of the cell from the external surroundings. It plays a vital role in maintaining cellular homeostasis through several mechanisms:
Selective Permeability: The plasma membrane regulates the entry and exit of substances, allowing essential nutrients (e.g., glucose, amino acids) to enter while preventing the influx of harmful substances. This selectivity is achieved through the lipid bilayer’s properties and the presence of specific transport proteins.
Transport Proteins:
- Channel Proteins: These proteins form pores that allow the passive movement of ions and small molecules down their concentration gradients.
- Carrier Proteins: These proteins undergo conformational changes to transport specific molecules across the membrane, either passively or actively.
- Pump Proteins: Active transporters, such as the sodium-potassium pump, use energy (ATP) to move ions against their concentration gradients, maintaining essential electrochemical gradients.
Signal Transduction: The plasma membrane contains receptors that detect and respond to extracellular signals (e.g., hormones, growth factors). This allows the cell to adjust its activities in response to environmental changes, contributing to homeostasis.
Cell Communication and Adhesion: The membrane facilitates interactions with other cells and the extracellular matrix through adhesion molecules and junctions, ensuring coordinated tissue function and structural integrity.
Fluidity and Flexibility: The lipid composition and presence of cholesterol maintain membrane fluidity, allowing the membrane to adapt to temperature changes and enabling the movement of membrane proteins as needed.
Ion Balance and Osmotic Pressure: By regulating ion channels and transporters, the plasma membrane maintains the proper balance of ions and osmotic pressure within the cell, preventing osmotic swelling or shrinkage.
Endocytosis and Exocytosis: These processes allow the cell to uptake large molecules and remove waste products, respectively, contributing to the dynamic maintenance of the cell’s internal environment.
Through these mechanisms, the plasma membrane ensures that the cell maintains a stable internal environment, essential for optimal function and survival.
Question 4:
Explain the process of active transport and provide an example of its role in cell physiology.
Answer:
Active transport is the movement of molecules or ions across a cell membrane from a region of lower concentration to a region of higher concentration, against their concentration gradient. This process requires energy, typically derived from adenosine triphosphate (ATP), and involves specific transport proteins known as pumps.
Key Features of Active Transport:
Energy-Dependent: Unlike passive transport, active transport requires energy input to move substances against their natural diffusion gradients.
Specificity: Transport proteins involved in active transport are highly specific to the molecules or ions they move, ensuring precise regulation of cellular concentrations.
Regulation: Active transport can be regulated by the cell to respond to changing physiological needs, maintaining homeostasis.
Example: Sodium-Potassium Pump (Na⁺/K⁺-ATPase):
The sodium-potassium pump is a quintessential example of active transport in cell physiology. Its primary roles include:
Maintaining Electrochemical Gradients: The pump moves three sodium ions (Na⁺) out of the cell and two potassium ions (K⁺) into the cell per ATP molecule consumed. This creates a high concentration of Na⁺ outside and a high concentration of K⁺ inside the cell.
Resting Membrane Potential: By maintaining differential ion concentrations, the pump contributes to the resting membrane potential, which is essential for functions such as nerve impulse transmission and muscle contraction.
Cell Volume Regulation: By controlling ion concentrations, the pump helps regulate osmotic balance and prevent excessive swelling or shrinkage of the cell.
Secondary Active Transport: The sodium gradient established by the pump drives the secondary active transport of other molecules, such as glucose and amino acids, into the cell via symporters.
Overall, active transport mechanisms like the sodium-potassium pump are vital for numerous physiological processes, ensuring that cells maintain the necessary internal environment to function correctly.
Question 5:
What is the role of lysosomes in cellular digestion and waste removal?
Answer:
Lysosomes are membrane-bound organelles containing hydrolytic enzymes responsible for breaking down various biomolecules. They play a crucial role in cellular digestion and waste removal through the following functions:
Intracellular Digestion:
Autophagy: Lysosomes degrade damaged or obsolete organelles and proteins within the cell. During autophagy, cellular components are encapsulated in autophagosomes, which then fuse with lysosomes for degradation and recycling of their constituents.
Endocytosis: Materials taken up by the cell through endocytosis, such as pathogens, extracellular proteins, or particulate matter, are delivered to lysosomes for breakdown and processing.
Extracellular Digestion:
- Phagocytosis: In specialized cells like macrophages, lysosomes fuse with phagosomes containing engulfed pathogens or debris, facilitating their destruction and clearance from the body.
Waste Removal:
Enzyme Degradation: Lysosomal enzymes break down waste materials and cellular debris into basic molecules (e.g., amino acids, sugars, fatty acids) that can be recycled or expelled from the cell.
Preventing Cellular Damage: By compartmentalizing degradative enzymes within lysosomes, cells prevent accidental damage to essential biomolecules and cellular structures.
Autolysis Prevention: Lysosomes contain a variety of enzymes capable of degrading cellular components. To prevent self-digestion, lysosomal membranes are robust and contain transport mechanisms to release only the necessary degradation products.
Role in Disease: Dysfunctional lysosomes can lead to the accumulation of undigested substrates, causing lysosomal storage diseases. Proper lysosomal function is essential for cellular health and overall organismal well-being.
In summary, lysosomes are integral to maintaining cellular homeostasis by efficiently managing the degradation and recycling of cellular waste and external materials, thereby ensuring the cell remains free of harmful debris and can recover valuable molecular components.
Question 6:
How do ion channels contribute to the electrical activity of neurons?
Answer:
Ion channels are integral membrane proteins that facilitate the selective passage of ions across the neuronal membrane, thereby contributing to the electrical activity essential for neuron function. Their roles in electrical activity include:
Generation of Action Potentials:
Resting Membrane Potential: Neurons maintain a resting membrane potential, typically around -70 mV, due to the differential distribution of ions (mainly Na⁺ and K⁺) and the selective permeability of ion channels.
Depolarization: When a neuron receives a stimulus, voltage-gated Na⁺ channels open, allowing Na⁺ to influx into the cell. This rapid influx causes the membrane potential to become less negative (depolarization).
Repolarization: Following depolarization, voltage-gated K⁺ channels open, allowing K⁺ to efflux out of the cell. This outflow restores the negative membrane potential (repolarization).
Hyperpolarization: The delayed closing of K⁺ channels can lead to a temporary hyperpolarization, making the membrane potential more negative than the resting state.
Propagation of Electrical Signals:
Action Potential Transmission: The sequential opening and closing of ion channels along the axon allow the action potential to propagate rapidly from the neuron’s cell body to its synaptic terminals.
Myelination and Saltatory Conduction: In myelinated neurons, ion channels are concentrated at the nodes of Ranvier, facilitating the jumping of action potentials between nodes and increasing conduction speed.
Synaptic Transmission:
Postsynaptic Potentials: Ion channels in the postsynaptic membrane respond to neurotransmitters released from the presynaptic neuron, leading to excitatory or inhibitory postsynaptic potentials that influence the likelihood of action potential generation.
Integration of Signals: The opening of various ion channels allows neurons to integrate multiple synaptic inputs, determining the overall electrical response and communication with other neurons.
Regulation of Neuronal Excitability: The types, distribution, and kinetics of ion channels determine the excitability of neurons, influencing how they respond to stimuli and communicate within neural networks.
In summary, ion channels are fundamental to the electrical signaling mechanisms of neurons, enabling the initiation, propagation, and modulation of action potentials that underlie all aspects of neural communication and function.
Question 7:
What mechanisms do cells use to regulate pH, and why is pH regulation important for cell physiology?
Answer:
Cells employ several mechanisms to regulate intracellular pH, maintaining it within a narrow range essential for optimal enzyme activity, metabolic processes, and overall cellular function. Key pH regulation mechanisms include:
Buffer Systems:
Bicarbonate Buffer System: This is the primary buffer in cells, involving the equilibrium between carbonic acid (H₂CO₃) and bicarbonate ions (HCO₃⁻). It helps neutralize excess hydrogen ions (H⁺) or hydroxide ions (OH⁻), minimizing pH fluctuations.
Phosphate Buffer System: Phosphate buffers, consisting of dihydrogen phosphate (H₂PO₄⁻) and hydrogen phosphate (HPO₄²⁻), provide additional buffering capacity, especially in intracellular environments.
Protein Buffers: Proteins, particularly those with amino acid side chains like histidine, can act as buffers by binding or releasing H⁺ ions in response to pH changes.
Ion Transport Mechanisms:
Proton Pumps: Proteins like the V-ATPase actively transport H⁺ ions out of the cytoplasm into organelles or the extracellular space, helping to lower intracellular H⁺ concentration and raise pH.
Exchangers and Cotransporters: Systems such as the sodium-hydrogen exchanger (NHE) remove H⁺ ions from the cell in exchange for Na⁺, while bicarbonate transporters facilitate the movement of bicarbonate ions to neutralize excess H⁺.
Respiratory Regulation:
Carbon Dioxide Removal: Through cellular respiration, cells produce CO₂, which can be converted to bicarbonate ions and excreted, thereby reducing the acid load and helping to regulate pH.
Mitochondrial Activity: Mitochondria help buffer cytoplasmic pH by consuming H⁺ ions during ATP production and exporting H⁺ as part of the proton gradient.
Metabolic Pathways:
Amino Acid Metabolism: The metabolism of amino acids can consume or produce H⁺ ions, contributing to pH balance.
Lactic Acid Fermentation: In anaerobic conditions, cells produce lactate and H⁺ ions, which need to be buffered and removed to prevent acidification.
Importance of pH Regulation:
Enzyme Activity: Most cellular enzymes have an optimal pH range. Deviations can lead to reduced enzyme activity or denaturation, impairing metabolic processes.
Metabolic Efficiency: Proper pH ensures the efficient functioning of metabolic pathways, including glycolysis, the citric acid cycle, and oxidative phosphorylation.
Structural Integrity: Proteins and nucleic acids maintain their structure and function within specific pH ranges. Altered pH can disrupt hydrogen bonds and ionic interactions, leading to structural changes.
Ion Transport and Membrane Potential: pH affects the charge and function of ion channels and transporters, influencing membrane potential and cellular excitability.
Signal Transduction: Many signaling pathways are sensitive to pH changes, which can modulate the activity of signaling proteins and pathways.
Maintaining pH homeostasis is thus critical for preserving cellular function, viability, and overall physiological health.
Question 8:
How do cells utilize ATP in various physiological processes? Provide specific examples.
Answer:
Adenosine triphosphate (ATP) serves as the primary energy currency in cells, powering a wide range of physiological processes. Cells utilize ATP through its ability to release energy upon hydrolysis (conversion to ADP and inorganic phosphate). Specific examples include:
Active Transport:
Sodium-Potassium Pump (Na⁺/K⁺-ATPase): ATP provides the energy required to transport Na⁺ out of and K⁺ into the cell against their concentration gradients, maintaining essential electrochemical gradients.
Calcium Pumps: ATP fuels the extrusion of Ca²⁺ from the cytoplasm into the endoplasmic reticulum or extracellular space, crucial for muscle contraction and signaling pathways.
Muscle Contraction:
Actin-Myosin Interaction: ATP binds to myosin heads, allowing them to detach from actin filaments after a power stroke. Hydrolysis of ATP to ADP and Pi repositions the myosin heads for another contraction cycle.
Cross-Bridge Cycling: The continuous binding and hydrolysis of ATP drive the repetitive cycles of muscle fiber contraction and relaxation.
Biosynthesis:
Protein Synthesis: ATP provides energy for the assembly of amino acids into polypeptide chains during translation.
Nucleic Acid Synthesis: DNA and RNA polymerases use ATP to add nucleotides to growing nucleic acid chains.
Cellular Signaling:
Phosphorylation Reactions: ATP donates phosphate groups to proteins and lipids in phosphorylation reactions, modulating their activity and enabling signal transduction pathways.
Second Messengers: ATP is a precursor for the synthesis of cyclic AMP (cAMP), a key second messenger in many signaling pathways.
Metabolic Pathways:
Glycolysis and the Citric Acid Cycle: ATP is both consumed and produced in these metabolic pathways, facilitating the breakdown of glucose and other nutrients for energy extraction.
Electron Transport Chain: ATP synthase utilizes the proton gradient generated by the electron transport chain to produce ATP from ADP and Pi.
Cell Division:
Chromosome Segregation: ATP powers motor proteins like kinesin and dynein, which are essential for the movement of chromosomes during mitosis.
Spindle Formation: ATP is required for the assembly and function of the mitotic spindle apparatus, ensuring accurate chromosome separation.
Maintenance of Cytoskeleton:
Microtubule Dynamics: ATP is involved in the polymerization and depolymerization of microtubules, crucial for maintaining cell shape and enabling intracellular transport.
Actin Filament Remodeling: ATP hydrolysis by actin-binding proteins regulates the assembly and disassembly of actin filaments, supporting cell motility and structure.
In summary, ATP is indispensable for powering diverse cellular activities, from active transport and muscle contraction to biosynthesis and signal transduction, underpinning the fundamental processes necessary for life.
Question 9:
What is the significance of the Golgi apparatus in protein sorting and trafficking within the cell?
Answer:
The Golgi apparatus is a central organelle involved in the modification, sorting, and trafficking of proteins and lipids within the cell. Its significance in protein sorting and trafficking includes:
Protein Modification:
Glycosylation: The Golgi modifies proteins by adding carbohydrate groups, which are essential for protein folding, stability, and cell signaling.
Phosphorylation and Sulfation: These modifications can alter protein activity, localization, and interactions with other molecules.
Proteolytic Processing: The Golgi may cleave precursor proteins into their active forms, regulating their functionality.
Protein Sorting:
Vesicle Formation: The Golgi packages modified proteins into vesicles based on their destination. This sorting ensures that proteins are delivered to the correct location, such as the plasma membrane, lysosomes, or secretion outside the cell.
Signal Recognition: Sorting signals within the protein’s structure are recognized by specific coat proteins (e.g., clathrin) that facilitate the formation of transport vesicles.
Trafficking Pathways:
Secretory Pathway: Proteins destined for secretion are transported from the Golgi to the plasma membrane via secretory vesicles, enabling their release into the extracellular environment.
Lysosomal Pathway: Enzymes and membrane proteins targeted for lysosomes are packaged into vesicles containing mannose-6-phosphate receptors, ensuring their delivery to lysosomes.
Endocytic Pathway: The Golgi also plays a role in recycling membrane proteins and lipids back to the plasma membrane or other organelles.
Membrane Lipid Composition:
Lipid Transport: The Golgi modifies lipid composition by adding or rearranging lipid molecules, which is essential for maintaining membrane fluidity and functionality.
Sphingolipid and Glycolipid Synthesis: These lipids are crucial for cell membrane structure and cell-cell communication.
Quality Control:
- Protein Folding Verification: The Golgi ensures that only properly folded and modified proteins are forwarded to their destinations. Misfolded proteins are typically targeted for degradation.
Cell Polarity and Function:
- Directed Secretion: In polarized cells (e.g., epithelial cells), the Golgi directs proteins to specific membrane domains, maintaining cell polarity and specialized functions.
Significance:
The Golgi apparatus is essential for ensuring that proteins and lipids are correctly processed, sorted, and delivered to their appropriate cellular locations. This precision is crucial for maintaining cellular organization, functionality, and communication. Disruptions in Golgi function can lead to diseases related to protein misfolding, trafficking defects, and impaired cellular signaling.
Question 10:
How do cells respond to changes in their external environment to maintain physiological balance?
Answer:
Cells employ a variety of mechanisms to detect and respond to changes in their external environment, ensuring the maintenance of physiological balance (homeostasis). These responses involve sensing, signal transduction, and appropriate cellular adjustments. Key strategies include:
Receptor-Mediated Signal Transduction:
Cell Surface Receptors: Cells have receptors on their plasma membrane (e.g., G-protein-coupled receptors, receptor tyrosine kinases) that detect extracellular signals like hormones, growth factors, and neurotransmitters.
Intracellular Receptors: Some signals (e.g., steroid hormones) pass through the membrane and bind to intracellular receptors, directly influencing gene expression.
Second Messengers:
Amplification of Signals: Binding of extracellular signals to receptors triggers the production of second messengers (e.g., cAMP, calcium ions, IP₃), which amplify and propagate the signal within the cell.
Activation of Kinases: Second messengers activate kinases that phosphorylate target proteins, altering their activity and leading to specific cellular responses.
Gene Expression Regulation:
Transcriptional Responses: Cells can upregulate or downregulate the expression of specific genes in response to environmental changes, producing proteins needed to adapt to new conditions.
Post-Transcriptional Modifications: mRNA stability and translation can be regulated to swiftly adjust protein synthesis in response to immediate needs.
Metabolic Adjustments:
Enzyme Activity Modulation: Cells can alter the activity of metabolic enzymes through allosteric regulation or covalent modification to meet changing energy demands or resource availability.
Pathway Shifts: Metabolic pathways can be rerouted to prioritize the synthesis of essential molecules or the breakdown of harmful substances.
Transport Mechanisms:
Ion Channel Regulation: Cells can adjust the opening and closing of ion channels to maintain ion homeostasis, respond to osmotic changes, and regulate membrane potential.
Vesicular Transport: The rate of endocytosis and exocytosis can be modulated to control the uptake of nutrients and the removal of waste products.
Cytoskeletal Remodeling:
Cell Shape Changes: The cytoskeleton can reorganize to facilitate cell movement, division, or changes in cell shape in response to environmental stimuli.
Intracellular Transport: Adjustments in the cytoskeleton support the directed movement of organelles and vesicles to adapt to new functional requirements.
Stress Response Pathways:
Heat Shock Proteins: In response to heat or other stressors, cells produce heat shock proteins that assist in protein folding and prevent aggregation.
Oxidative Stress Response: Cells upregulate antioxidant enzymes to neutralize reactive oxygen species generated under stress conditions.
Feedback Mechanisms:
Negative Feedback: Cells use negative feedback loops to dampen responses once homeostasis is restored, preventing overcompensation.
Positive Feedback: In certain cases, positive feedback amplifies responses to achieve a rapid or decisive change, such as during the initiation of an action potential.
Apoptosis:
- Programmed Cell Death: In cases of severe stress or irreparable damage, cells may undergo apoptosis to eliminate dysfunctional cells and protect the organism.
Importance of Responsive Adaptation:
By effectively responding to environmental changes, cells can maintain internal stability, ensure proper functioning, and survive under varying conditions. This adaptability is crucial for the health of individual cells and the overall organism, allowing for growth, repair, and defense against external threats.
Question 11:
Discuss the role of peroxisomes in cellular metabolism and detoxification.
Answer:
Peroxisomes are small, membrane-bound organelles involved in various aspects of cellular metabolism and detoxification. Their roles include:
Fatty Acid β-Oxidation:
Breakdown of Very Long-Chain Fatty Acids: Peroxisomes degrade very long-chain fatty acids (VLCFAs) through β-oxidation, shortening them to medium-chain fatty acids that can be further processed in mitochondria.
Diverse Substrate Specificity: Unlike mitochondrial β-oxidation, peroxisomal β-oxidation can process a broader range of fatty acid substrates, including branched-chain and unsaturated fatty acids.
Detoxification of Harmful Metabolites:
Hydrogen Peroxide Degradation: Peroxisomes contain catalase, an enzyme that breaks down hydrogen peroxide (H₂O₂), a reactive oxygen species, into water and oxygen, preventing oxidative damage to cellular components.
Metabolism of Reactive Oxygen Species: Peroxisomes help manage reactive oxygen species (ROS), maintaining cellular redox balance and protecting against oxidative stress.
Biosynthesis of Plasmalogens:
- Phospholipid Synthesis: Peroxisomes are involved in the synthesis of plasmalogens, a type of phospholipid essential for the normal function of the brain and heart, as well as for maintaining the integrity of cell membranes.
Metabolism of Amino Acids and Polyamines:
- Degradation Pathways: Peroxisomes participate in the catabolism of certain amino acids and polyamines, contributing to nitrogen balance and the production of metabolites used in other cellular processes.
Metabolism of Bile Acids:
- Synthesis and Breakdown: In liver cells, peroxisomes are involved in the synthesis and breakdown of bile acids, which are critical for the digestion and absorption of dietary fats.
Steroid Metabolism:
- Conversion Processes: Peroxisomes assist in the metabolism of steroids, including the synthesis and degradation of steroid hormones, influencing various physiological functions.
Lipid Elongation and Desaturation:
- Fatty Acid Modification: Peroxisomes contribute to the elongation and desaturation of fatty acids, modifying them to meet specific cellular needs.
Role in Cellular Detoxification:
Peroxisomes play a vital role in detoxifying harmful substances:
Breakdown of Xenobiotics: They degrade toxic compounds, including alcohols and aldehydes, preventing their accumulation and potential damage.
Reduction of Oxidative Stress: By neutralizing hydrogen peroxide and other ROS, peroxisomes protect cellular components from oxidative damage, which can lead to mutations, protein dysfunction, and lipid peroxidation.
Clinical Significance:
Defects in peroxisomal function can lead to metabolic disorders, such as Zellweger syndrome and adrenoleukodystrophy, characterized by the accumulation of VLCFAs and other toxic metabolites. These conditions often result in severe developmental and neurological abnormalities, highlighting the essential role of peroxisomes in cellular and organismal health.
In summary, peroxisomes are indispensable for lipid metabolism, detoxification of harmful substances, and maintenance of cellular homeostasis, underscoring their critical role in cell physiology.
Question 12:
How do cells utilize the cytoskeleton to facilitate intracellular transport and maintain cell shape?
Answer:
The cytoskeleton is a dynamic network of protein fibers that provides structural support, facilitates intracellular transport, and maintains cell shape. It consists of three main types of filaments: microfilaments (actin filaments), intermediate filaments, and microtubules. Each component plays distinct roles in cellular functions:
Maintaining Cell Shape:
Microfilaments (Actin Filaments): These thin, flexible fibers form a dense network beneath the plasma membrane, contributing to the cell’s mechanical strength and enabling changes in shape during processes like migration and division.
Intermediate Filaments: Providing tensile strength, intermediate filaments stabilize the cell’s structure and anchor organelles, ensuring resilience against mechanical stress.
Microtubules: These rigid, hollow tubes maintain cell shape by resisting compression and providing a framework that supports organelle positioning and the overall architecture of the cell.
Intracellular Transport:
Motor Proteins: Proteins such as kinesin and dynein move along microtubules, carrying vesicles, organelles, and other cargoes to specific cellular destinations. Myosin motors interact with actin filaments to transport materials within the cell.
Vesicle Trafficking: The cytoskeleton guides vesicles through the cytoplasm, ensuring accurate delivery to target membranes for processes like exocytosis and endocytosis.
Organelle Positioning: Microtubules help position organelles like the Golgi apparatus, endoplasmic reticulum, and mitochondria within the cell, optimizing their function and interactions.
Cell Division:
Mitotic Spindle Formation: Microtubules form the spindle apparatus, which segregates chromosomes during mitosis, ensuring equal distribution to daughter cells.
Cytokinesis: Actin filaments and myosin motors contract to form the cleavage furrow, dividing the cytoplasm between daughter cells.
Cell Motility:
Amoeboid Movement: Actin polymerization and depolymerization drive protrusions like pseudopodia, enabling cells to move and navigate their environment.
Cilia and Flagella: Microtubule-based structures generate coordinated movements for cell locomotion and the movement of fluids across cell surfaces.
Signal Transduction and Cellular Communication:
Anchoring Signaling Complexes: The cytoskeleton anchors various signaling molecules, facilitating efficient signal transduction and communication within the cell.
Mechanical Signal Transduction: The cytoskeleton senses mechanical forces and transduces them into biochemical signals, influencing cellular responses like differentiation and proliferation.
Spatial Organization of Cellular Components:
- Compartmentalization: The cytoskeleton helps organize the spatial distribution of organelles and protein complexes, optimizing cellular functions and interactions.
Dynamic Nature of the Cytoskeleton:
The cytoskeleton is highly dynamic, continuously undergoing polymerization and depolymerization to respond to cellular needs. This plasticity allows cells to adapt their shape, migrate, divide, and transport materials efficiently.
Clinical Relevance:
Disruptions in cytoskeletal components or their regulation can lead to various diseases, including neurodegenerative disorders, muscular dystrophies, and cancer metastasis. Understanding cytoskeletal dynamics is essential for developing therapeutic strategies targeting these conditions.
In conclusion, the cytoskeleton is integral to maintaining cell structure, enabling intracellular transport, facilitating cell movement, and ensuring proper cell division, all of which are essential for the proper functioning and survival of cells.
Cell Physiology and Biochemistry: Thought-Provoking Questions
1. How do mitochondria and chloroplasts collaborate to maintain cellular energy balance in eukaryotic cells?
Answer: Mitochondria and chloroplasts are essential organelles involved in energy metabolism in eukaryotic cells. While mitochondria are responsible for cellular respiration in both plant and animal cells, chloroplasts are exclusive to plant cells and certain algae, facilitating photosynthesis. Their collaboration ensures a balanced energy state within the cell through the following mechanisms:
- Energy Production and Consumption:
- Chloroplasts: During photosynthesis, chloroplasts convert light energy into chemical energy in the form of glucose and other carbohydrates. This process also generates oxygen as a byproduct.
- Mitochondria: Mitochondria utilize the glucose produced by chloroplasts to generate ATP through oxidative phosphorylation during cellular respiration. This ATP serves as the primary energy currency for various cellular processes.
- Metabolic Integration:
- The glucose synthesized in chloroplasts enters the glycolytic pathway, producing pyruvate that is transported into mitochondria. Here, it undergoes the citric acid cycle and oxidative phosphorylation to produce ATP.
- The oxygen produced by chloroplasts is consumed by mitochondria during cellular respiration, completing the cycle of oxygen utilization and carbon dioxide production.
- Regulation of Redox Balance:
- Both organelles participate in maintaining the cellular redox state. Photosynthesis in chloroplasts generates reducing equivalents (NADPH), which are used in various biosynthetic pathways. Mitochondria help balance these reducing agents by oxidizing NADH produced during respiration, ensuring that the cell remains in a state of redox equilibrium.
- Signal Transduction and Cellular Communication:
- Mitochondria and chloroplasts communicate through signaling molecules and metabolic intermediates. For example, the energy status of the cell, reflected by ATP and AMP levels, can influence the activity of both organelles, coordinating their functions to meet the cell’s energy demands.
- Adaptation to Environmental Changes:
- In response to fluctuating light conditions, chloroplasts adjust the rate of photosynthesis, while mitochondria modulate cellular respiration to optimize ATP production. This dynamic interplay ensures that cells efficiently manage energy resources under varying environmental conditions.
In summary, mitochondria and chloroplasts work in tandem to convert and manage energy within eukaryotic cells. Chloroplasts capture and store energy from light, while mitochondria release and utilize this energy to produce ATP, maintaining cellular energy balance and supporting diverse physiological functions.
2. In what ways do ion gradients across the plasma membrane influence cellular processes such as nerve impulse transmission and muscle contraction?
Answer: Ion gradients across the plasma membrane are fundamental to various cellular processes, particularly in excitable cells like neurons and muscle cells. These gradients are established and maintained by ion pumps and channels, creating differences in ion concentrations and electrical charge across the membrane. Their influence on nerve impulse transmission and muscle contraction includes:
- Resting Membrane Potential:
- Neurons and muscle cells maintain a resting membrane potential, typically around -70 mV, primarily due to the distribution of ions like Na⁺, K⁺, Cl⁻, and negatively charged proteins inside the cell. The sodium-potassium pump (Na⁺/K⁺-ATPase) actively transports 3 Na⁺ ions out and 2 K⁺ ions into the cell, contributing to the negative internal charge.
- Nerve Impulse Transmission:
- Action Potential Generation: When a neuron is stimulated, voltage-gated Na⁺ channels open, allowing Na⁺ to rush into the cell, causing rapid depolarization. This is followed by the opening of voltage-gated K⁺ channels, allowing K⁺ to exit, repolarizing the membrane.
- Propagation of the Impulse: The sequential opening and closing of ion channels along the axon create a wave of depolarization, propagating the action potential toward the synaptic terminal.
- Refractory Periods: The inactivation of Na⁺ channels and delayed closing of K⁺ channels create refractory periods, ensuring the unidirectional transmission of the nerve impulse and preventing the backward flow of the action potential.
- Muscle Contraction:
- Excitation-Contraction Coupling: In muscle cells, an action potential travels along the sarcolemma and down the T-tubules, triggering the release of Ca²⁺ from the sarcoplasmic reticulum.
- Calcium’s Role: The increase in intracellular Ca²⁺ binds to troponin, causing a conformational change that moves tropomyosin away from actin-binding sites, allowing myosin to bind actin and initiate the cross-bridge cycle leading to contraction.
- Relaxation: Ca²⁺ is actively pumped back into the sarcoplasmic reticulum by Ca²⁺-ATPase pumps, decreasing intracellular Ca²⁺ levels and allowing muscle fibers to relax.
- Signal Transduction and Cellular Communication:
- Neurotransmitter Release: The influx of Ca²⁺ into the presynaptic terminal via voltage-gated Ca²⁺ channels triggers the fusion of synaptic vesicles with the plasma membrane, releasing neurotransmitters into the synaptic cleft.
- Energy Consumption and Ion Homeostasis:
- Maintaining ion gradients requires ATP, primarily consumed by the Na⁺/K⁺-ATPase pump. This ensures that the gradients are preserved, enabling continuous nerve impulse transmission and muscle contractions.
- Adaptation and Plasticity:
- Neurons can adjust the density and sensitivity of ion channels based on activity levels, contributing to neural plasticity and the ability to adapt to new information or stimuli.
In essence, ion gradients across the plasma membrane are crucial for the electrical excitability of neurons and muscle cells. They enable the rapid and controlled changes in membrane potential necessary for nerve impulses and muscle contractions, underpinning fundamental physiological processes such as movement, sensation, and communication within the nervous system.
3. How does the structure of the plasma membrane facilitate its role in selective permeability and signal transduction?
Answer: The plasma membrane’s structure is intricately designed to fulfill its roles in selective permeability and signal transduction through several key features:
- Lipid Bilayer Composition:
- Phospholipid Arrangement: The plasma membrane consists of a double layer of phospholipids, with hydrophilic heads facing outward toward the aqueous environment and hydrophobic tails facing inward. This arrangement creates a semi-permeable barrier that allows selective passage of molecules based on size, polarity, and solubility.
- Fluid Mosaic Model: The membrane is fluid, allowing lateral movement of lipids and proteins. This fluidity is essential for the dynamic functions of the membrane, including the formation of lipid rafts and the movement of signaling complexes.
- Integral and Peripheral Proteins:
- Transport Proteins: Channel proteins and carrier proteins embedded within the lipid bilayer facilitate the selective transport of ions and molecules, ensuring that only specific substances enter or exit the cell.
- Receptor Proteins: Transmembrane receptors bind extracellular signaling molecules (ligands) and initiate intracellular signal transduction pathways. Their specific binding ensures precise cellular responses to external stimuli.
- Cholesterol Molecules:
- Membrane Fluidity Regulation: Cholesterol molecules interspersed within the lipid bilayer modulate membrane fluidity, maintaining its integrity across varying temperatures and preventing it from becoming too rigid or too fluid.
- Stabilization of Protein Function: Cholesterol can influence the conformation and function of membrane proteins, affecting their role in signal transduction and transport.
- Carbohydrate Chains:
- Cell Recognition and Adhesion: Glycoproteins and glycolipids on the extracellular surface of the membrane play roles in cell-cell recognition, adhesion, and communication, essential for tissue formation and immune responses.
- Signal Modulation: Carbohydrate chains can modulate receptor activity and ligand binding, influencing the strength and duration of signaling events.
- Membrane Microdomains:
- Lipid Rafts: Specialized microdomains enriched in cholesterol and sphingolipids organize signaling molecules, facilitating efficient signal transduction by bringing together receptors, kinases, and other signaling proteins.
- Protein Complexes: The clustering of proteins within these microdomains enhances the specificity and speed of signal transduction pathways.
- Asymmetrical Distribution:
- Functional Specialization: The different compositions of the inner and outer leaflets of the lipid bilayer allow for specialized functions. For example, certain phospholipids are predominantly found on the inner side, interacting with the cytoskeleton and intracellular signaling proteins.
- Dynamic Remodeling:
- Endocytosis and Exocytosis: The plasma membrane can rapidly change its composition and shape through vesicular transport processes, allowing cells to adapt to changing environments and regulate the availability of receptors and transporters.
- Integration of Structural and Signaling Functions:
- Cytoskeletal Interactions: The cytoskeleton is connected to the plasma membrane through linker proteins, enabling the transmission of mechanical signals and the stabilization of membrane structures necessary for effective signal transduction.
- Signal Amplification:
- Multiple Binding Sites: Receptor proteins often have multiple binding sites for ligands, allowing for the amplification of signals as one ligand binding event can trigger multiple downstream signaling molecules.
- Temporal and Spatial Regulation:
- Receptor Recycling and Degradation: The membrane can regulate the number of active receptors through processes like endocytosis, recycling, or degradation, ensuring precise control over signal transduction intensity and duration.
In summary, the plasma membrane’s structural features—such as the lipid bilayer, embedded proteins, cholesterol content, carbohydrate chains, and dynamic organization—are meticulously arranged to facilitate selective permeability and efficient signal transduction. These structural attributes enable cells to interact with their environment, respond to external signals, and maintain internal homeostasis with remarkable precision and adaptability.
4. What role do motor proteins play in intracellular transport, and how do defects in these proteins contribute to human diseases?
Answer: Motor proteins are essential for intracellular transport, facilitating the movement of organelles, vesicles, and other cargoes along the cytoskeletal networks of the cell. They convert chemical energy from ATP hydrolysis into mechanical work, enabling the directed transport necessary for various cellular functions. The primary motor proteins include kinesins, dyneins, and myosins, each associated with specific cytoskeletal filaments:
Types of Motor Proteins:
- Kinesins: Typically move cargoes toward the plus (+) end of microtubules, usually directed towards the cell periphery. They are involved in transporting vesicles, organelles like mitochondria, and protein complexes.
- Dyneins: Move cargoes toward the minus (-) end of microtubules, generally towards the cell center. They are crucial for retrograde transport, moving vesicles and organelles back to the Golgi apparatus and the nucleus.
- Myosins: Associated with actin filaments, myosins are involved in transporting cargoes within the cell, muscle contraction, and cell motility.
Functions in Intracellular Transport:
- Vesicle Trafficking: Motor proteins transport vesicles between the endoplasmic reticulum, Golgi apparatus, lysosomes, and the plasma membrane, ensuring proper distribution of proteins and lipids.
- Organelle Positioning: They help position organelles like the nucleus, mitochondria, and peroxisomes within the cell, maintaining cellular organization and function.
- Signal Transduction: Transport of signaling molecules and receptors to specific cellular locations is mediated by motor proteins, enabling efficient signal transduction pathways.
- Cell Division: During mitosis, motor proteins are involved in the formation and function of the mitotic spindle, ensuring accurate chromosome segregation.
Impact of Defects in Motor Proteins:
- Neurodegenerative Diseases:
- Huntington’s Disease: Caused by defects in the motor protein dynein, leading to impaired axonal transport and the accumulation of toxic proteins in neurons.
- Amyotrophic Lateral Sclerosis (ALS): Mutations in motor proteins like dynein and kinesin disrupt the transport of essential components along axons, contributing to motor neuron degeneration.
- Charcot-Marie-Tooth Disease: Mutations in myosin proteins affect the transport of vesicles and organelles in peripheral nerves, leading to muscle weakness and atrophy.
- Muscular Dystrophies:
- Myosin Defects: Mutations in myosin proteins can impair muscle contraction and maintenance, resulting in muscle wasting and weakness seen in various muscular dystrophies.
- Ciliopathies:
- Dynein Mutations: Affect the function of cilia and flagella, leading to disorders such as primary ciliary dyskinesia, which causes respiratory issues and infertility.
- Cancer:
- Kinesin Overexpression: Abnormal expression of certain kinesins can lead to uncontrolled cell division and tumor progression by disrupting mitotic spindle function.
- Cellular Transport Disorders:
- Cargo Accumulation: Defects in motor proteins can cause the accumulation of vesicles and organelles, disrupting cellular homeostasis and leading to cell dysfunction or death.
- Developmental Abnormalities:
- Impaired Organogenesis: Motor protein defects can disrupt the transport of signaling molecules and organelles during embryonic development, leading to congenital malformations.
- Neurodegenerative Diseases:
Therapeutic Implications:
- Understanding motor protein defects has led to the development of targeted therapies aimed at restoring proper intracellular transport. For example, small molecules that enhance motor protein function or compensate for their deficits are being explored as potential treatments for neurodegenerative diseases.
In summary, motor proteins are indispensable for the precise and efficient transport of cellular components. Defects in these proteins disrupt intracellular logistics, leading to a cascade of cellular dysfunctions that manifest as various human diseases, particularly those affecting the nervous and muscular systems. Continued research into motor protein mechanisms and their role in disease offers promising avenues for therapeutic intervention.
5. How does the process of endocytosis contribute to nutrient uptake and receptor-mediated signaling in cells?
Answer: Endocytosis is a cellular process by which cells internalize extracellular materials, including nutrients, signaling molecules, and plasma membrane components. It plays a critical role in nutrient uptake and receptor-mediated signaling through the following mechanisms:
Types of Endocytosis:
- Phagocytosis: Engulfing large particles, such as pathogens or cellular debris, typically performed by specialized cells like macrophages.
- Pinocytosis: Uptake of extracellular fluid and dissolved solutes through small vesicles, commonly referred to as “cell drinking.”
- Receptor-Mediated Endocytosis: Highly specific internalization of molecules bound to cell surface receptors, enabling selective uptake and signal transduction.
Nutrient Uptake:
- Receptor-Mediated Endocytosis for Nutrients:
- Transferrin and Iron Uptake: Cells use transferrin receptors to specifically bind transferrin-bound iron. The transferrin-receptor complex is internalized via clathrin-coated pits, allowing cells to acquire essential iron for various metabolic processes.
- Low-Density Lipoprotein (LDL) Uptake: LDL receptors bind LDL particles carrying cholesterol. The LDL-receptor complex is endocytosed, delivering cholesterol to cells for membrane synthesis and steroid hormone production.
- Glucose Transport: Although glucose uptake primarily occurs through facilitated diffusion via GLUT transporters, certain specialized transport mechanisms can involve endocytosis for bulk nutrient uptake under specific conditions.
- Receptor-Mediated Endocytosis for Nutrients:
Receptor-Mediated Signaling:
- Signal Termination: After ligand binding, receptors can be internalized through endocytosis, removing them from the cell surface and attenuating the signaling response. This helps regulate the intensity and duration of signaling pathways.
- Signal Transduction from Endosomes: Some receptors continue to signal after internalization. For example, the epidermal growth factor receptor (EGFR) can activate downstream signaling pathways from endosomal compartments, contributing to sustained or spatially restricted signaling.
- Receptor Recycling and Degradation: Endocytosed receptors can be sorted for recycling back to the plasma membrane or directed to lysosomes for degradation. Recycling allows cells to reuse receptors for future signaling, while degradation prevents overstimulation and maintains signaling homeostasis.
Vesicular Transport of Signaling Molecules:
- Internalization of Ligand-Protein Complexes: Endocytosis can transport signaling molecules and their receptors into the cell, facilitating the activation of intracellular pathways and coordinating complex cellular responses.
Adaptation to Environmental Changes:
- Modulation of Receptor Density: Cells can adjust the number of receptors on their surface through endocytosis and recycling, allowing them to respond dynamically to varying concentrations of signaling molecules.
Nutrient Storage and Release:
- Vesicle Formation: Endocytosis can lead to the formation of vesicles that store nutrients or other essential molecules, releasing them when needed through exocytosis.
Pathogen Entry and Immune Response:
- Phagocytosis of Pathogens: Immune cells utilize phagocytosis to internalize and destroy invading microorganisms, contributing to the body’s defense mechanisms.
Cellular Homeostasis:
- Removal of Damaged Proteins and Membranes: Endocytosis helps eliminate damaged proteins and membrane fragments, maintaining cellular integrity and function.
Therapeutic Applications:
- Drug Delivery: Understanding endocytosis mechanisms facilitates the development of targeted drug delivery systems, enhancing the efficacy and specificity of therapeutics.
In conclusion, endocytosis is indispensable for the selective uptake of nutrients and the precise regulation of receptor-mediated signaling. By internalizing essential molecules and modulating receptor availability, endocytosis ensures that cells maintain metabolic balance, respond appropriately to external signals, and adapt to changing environmental conditions. Its multifaceted roles underscore its importance in cellular physiology and its potential as a target for therapeutic interventions.
6. Describe how calcium ions function as secondary messengers in cellular signaling pathways and their impact on cellular functions.
Answer: Calcium ions (Ca²⁺) are versatile secondary messengers in numerous cellular signaling pathways, playing pivotal roles in regulating a wide array of cellular functions. Their functionality as secondary messengers is characterized by their ability to rapidly and reversibly interact with various proteins to propagate and modulate signals initiated by primary messengers (e.g., hormones, neurotransmitters). The impact of Ca²⁺ on cellular functions includes:
Mechanism of Action as Secondary Messengers:
- Signal Initiation: Primary messengers bind to cell surface receptors, triggering the release of Ca²⁺ from intracellular stores such as the endoplasmic reticulum (ER) or influx from the extracellular environment through plasma membrane channels.
- Calcium Signaling Dynamics: The concentration of Ca²⁺ is tightly regulated. Upon signal activation, Ca²⁺ levels transiently increase in the cytoplasm, facilitating specific interactions with target proteins. Subsequently, Ca²⁺ is sequestered back into stores or expelled from the cell to terminate the signal.
Regulation of Enzymatic Activity:
- Calmodulin Activation: Ca²⁺ binds to calmodulin, a calcium-binding messenger protein. The Ca²⁺-calmodulin complex activates various kinases and phosphatases, modulating enzymatic activities involved in processes like metabolism, gene expression, and cytoskeletal rearrangement.
- Protein Kinase C (PKC): Certain isoforms of PKC require Ca²⁺ for activation. Once activated, PKC phosphorylates target proteins, influencing cell growth, differentiation, and apoptosis.
Gene Expression Regulation:
- NFAT Transcription Factors: Ca²⁺ signaling activates calcineurin, a phosphatase that dephosphorylates NFAT (nuclear factor of activated T-cells). Dephosphorylated NFAT translocates to the nucleus, promoting the transcription of genes involved in immune responses and other cellular processes.
Muscle Contraction:
- Excitation-Contraction Coupling: In muscle cells, Ca²⁺ released from the sarcoplasmic reticulum binds to troponin, inducing conformational changes that allow actin-myosin interactions, leading to muscle contraction.
Neurotransmitter Release:
- Synaptic Transmission: In neurons, the influx of Ca²⁺ into the presynaptic terminal upon action potential arrival triggers the fusion of synaptic vesicles with the plasma membrane, facilitating the release of neurotransmitters into the synaptic cleft.
Cell Motility and Cytoskeletal Dynamics:
- Actin Remodeling: Ca²⁺ regulates the activity of actin-binding proteins, such as gelsolin and cofilin, which modulate actin filament assembly and disassembly, thereby influencing cell shape and movement.
Apoptosis and Cell Survival:
- Mitochondrial Function: Elevated cytoplasmic Ca²⁺ can lead to mitochondrial dysfunction and the release of pro-apoptotic factors like cytochrome c, initiating programmed cell death.
- Survival Pathways: Conversely, controlled Ca²⁺ signaling can activate survival pathways by modulating kinase activities and gene expression.
Secretion and Exocytosis:
- Hormone Release: In endocrine cells, Ca²⁺ influx is crucial for the exocytosis of hormone-containing vesicles, ensuring the timely release of signaling molecules into the bloodstream.
Oocyte Activation and Fertilization:
- Calcium Waves: Fertilization triggers a series of Ca²⁺ oscillations in the oocyte, essential for egg activation and the initiation of embryonic development.
Immune Cell Activation:
- T-Cell Activation: In immune cells, Ca²⁺ signaling is critical for the activation of T-cells, influencing their proliferation and cytokine production during immune responses.
Homeostatic Regulation:
- Calcium Pumps and Exchangers: Cells utilize ATP-dependent pumps (e.g., SERCA) and exchangers (e.g., NCX) to maintain low cytoplasmic Ca²⁺ levels, ensuring that Ca²⁺ signaling is tightly controlled and localized.
Clinical Implications:
- Cardiac Function: Abnormal Ca²⁺ signaling in cardiac cells can lead to arrhythmias and heart failure.
- Neurodegenerative Diseases: Dysregulated Ca²⁺ homeostasis is implicated in conditions like Alzheimer’s disease and Parkinson’s disease, where excessive Ca²⁺ can contribute to neuronal death.
In summary, calcium ions serve as pivotal secondary messengers in a multitude of cellular signaling pathways. Their ability to interact with diverse target proteins and modulate various cellular processes underscores their importance in maintaining cellular function, responding to external stimuli, and ensuring proper physiological responses. The precise regulation of Ca²⁺ signaling is thus essential for cellular health and organismal homeostasis.
7. How do cells maintain pH homeostasis, and why is this regulation critical for enzymatic activity and metabolic processes?
Answer: Cells maintain pH homeostasis through a combination of buffering systems, active transport mechanisms, and metabolic processes. Maintaining an optimal intracellular pH is crucial for the proper functioning of enzymes, metabolic pathways, and overall cellular integrity. Here’s how cells achieve pH regulation and why it is essential:
Buffering Systems:
- Bicarbonate Buffer System: The primary buffer in cells, involving the equilibrium between carbonic acid (H₂CO₃) and bicarbonate ions (HCO₃⁻). This system neutralizes excess hydrogen ions (H⁺) or hydroxide ions (OH⁻), minimizing pH fluctuations.
- Phosphate Buffer System: Utilizes dihydrogen phosphate (H₂PO₄⁻) and hydrogen phosphate (HPO₄²⁻) to buffer intracellular pH, especially important in the cytoplasm.
- Protein Buffers: Amino acid residues in proteins, particularly those with side chains like histidine, act as buffers by binding or releasing H⁺ ions in response to pH changes.
Active Transport Mechanisms:
- Proton Pumps: Transport H⁺ ions out of the cytoplasm into organelles or the extracellular space, reducing cytoplasmic acidity. Examples include the V-ATPase pumps in lysosomes and the plasma membrane.
- Sodium-Hydrogen Exchangers (NHE): Exchange intracellular H⁺ for extracellular Na⁺, helping to expel excess H⁺ and regulate pH.
- Bicarbonate Transporters: Facilitate the movement of HCO₃⁻ ions to buffer cytoplasmic H⁺ concentrations.
Respiratory Regulation:
- CO₂ Removal: Cellular respiration produces CO₂, which can diffuse out of the cell and be exhaled by the lungs. This helps prevent the accumulation of carbonic acid, maintaining pH balance.
- Carbonic Anhydrase: Enzyme that catalyzes the conversion of CO₂ and water to carbonic acid, facilitating rapid pH regulation in response to metabolic changes.
Metabolic Pathways:
- Glycolysis and Citric Acid Cycle: Metabolic pathways produce and consume H⁺ ions. For instance, glycolysis generates pyruvate and NADH, while the citric acid cycle produces CO₂, which can be buffered by bicarbonate ions.
- Amino Acid Metabolism: Metabolism of amino acids can produce or consume H⁺ ions, contributing to pH regulation.
Cellular Organelles:
- Lysosomes: Contain acidic environments maintained by proton pumps, aiding in the breakdown of biomolecules. They also help regulate cytoplasmic pH by sequestering H⁺ ions.
- Mitochondria: Involved in ATP production and the consumption of H⁺ ions during oxidative phosphorylation, indirectly influencing cytoplasmic pH.
Osmotic Regulation:
- Ion Balance: Proper regulation of ion concentrations affects osmotic pressure, indirectly influencing pH by controlling the movement of H⁺ and other ions.
Importance of pH Regulation:
Enzymatic Activity:
- Optimal pH Range: Enzymes have specific pH optima where their catalytic activity is maximized. Deviations from this range can lead to reduced enzyme activity or denaturation.
- Structural Integrity: pH affects the ionization states of amino acid residues in enzyme active sites, influencing substrate binding and catalysis.
Metabolic Processes:
- Reaction Rates: pH changes can alter the rates of biochemical reactions by affecting enzyme kinetics and the availability of substrates and cofactors.
- Pathway Integration: Many metabolic pathways are interconnected, and pH changes in one pathway can influence others, disrupting overall metabolic balance.
Protein Function and Stability:
- Conformational Changes: Proteins rely on hydrogen bonds and ionic interactions for their three-dimensional structures. Altered pH can disrupt these interactions, leading to structural instability.
- Protein-Protein Interactions: pH affects the binding affinities between proteins, influencing complex formation and signaling pathways.
Ion Transport and Membrane Potential:
- Transporter Activity: Many ion transporters and channels are sensitive to pH, affecting their function and the maintenance of electrochemical gradients.
- Membrane Potential: pH influences the distribution of ions across the membrane, impacting the resting membrane potential and cellular excitability.
Cellular Communication:
- Signal Transduction: pH changes can modulate the activity of signaling proteins and transcription factors, influencing gene expression and cellular responses.
Homeostasis and Survival:
- Cellular Integrity: Extreme pH shifts can lead to cellular damage, apoptosis, or necrosis, threatening cell survival and tissue integrity.
- Adaptation to Stress: Proper pH regulation allows cells to adapt to metabolic stress, hypoxia, and other environmental challenges.
In conclusion, pH homeostasis is vital for maintaining the structural and functional integrity of cellular components, ensuring optimal enzyme activity, efficient metabolic processes, and overall cellular health. Cells employ a combination of buffering systems, active transport mechanisms, and metabolic adjustments to tightly regulate pH, underscoring its critical role in sustaining life.
8. How does the structure of tight junctions contribute to their function in maintaining the selective barrier of epithelial tissues?
Answer: Tight junctions are specialized intercellular connections found predominantly in epithelial and endothelial tissues. Their structure is intricately designed to fulfill their role in maintaining selective barriers, controlling the passage of ions, molecules, and cells between neighboring cells. Here’s how their structure contributes to their function:
Molecular Composition:
- Claudins: A family of transmembrane proteins that form the backbone of tight junction strands. Different claudin isoforms determine the permeability properties of the junctions, allowing selective passage based on size and charge.
- Occludin: Another transmembrane protein that contributes to the barrier function by interacting with claudins and the cytoskeleton. Its phosphorylation state can regulate tight junction assembly and permeability.
- JAMs (Junctional Adhesion Molecules): Immunoglobulin-like proteins that mediate homophilic and heterophilic interactions between adjacent cells, enhancing cell-cell adhesion and tightness.
Structural Organization:
- Zonula Occludens: Tight junctions are located at the apical-most region of the lateral membrane, forming a continuous belt-like structure encircling epithelial cells. This positioning ensures that they effectively seal the paracellular space.
- Fibril Networks: Tight junction proteins link to intracellular scaffolding proteins such as ZO-1, ZO-2, and ZO-3 (zonula occludens proteins). These scaffolds connect tight junctions to the actin cytoskeleton, providing structural support and facilitating dynamic regulation.
Paracellular Barrier Formation:
- Seal Formation: The interaction of claudins and occludin between adjacent cells creates a tight seal that restricts the free passage of solutes and water through the paracellular route. This seal is crucial for maintaining distinct extracellular environments on either side of the epithelial layer.
- Selective Permeability: Different claudin isoforms can create charge-selective pores or tight seals, allowing selective permeability. For instance, some claudins form channels that preferentially allow ions like Na⁺ or Cl⁻ to pass, contributing to tissue-specific ion transport and homeostasis.
Dynamic Regulation:
- Cytoskeletal Interactions: The connection to the actin cytoskeleton allows tight junctions to respond to mechanical stress and signaling cues. Actin remodeling can modulate tight junction integrity and permeability in response to physiological needs.
- Post-Translational Modifications: Phosphorylation, ubiquitination, and other modifications of tight junction proteins can regulate their assembly, disassembly, and function, enabling rapid adjustments to barrier properties during processes like inflammation, wound healing, and tissue remodeling.
Functional Implications:
- Maintaining Cell Polarity: Tight junctions prevent the mixing of apical and basolateral membrane proteins, maintaining epithelial cell polarity essential for directional transport and specialized functions.
- Preventing Pathogen Entry: By forming a robust barrier, tight junctions limit the access of pathogens and toxins to underlying tissues, contributing to the body’s defense mechanisms.
- Regulating Ion Transport: In tissues like the kidney and intestines, tight junctions control ion permeability, facilitating selective absorption and secretion processes critical for electrolyte balance and fluid homeostasis.
Clinical Relevance:
- Barrier Dysfunction: Disruption of tight junctions is implicated in various diseases, including inflammatory bowel disease, asthma, and certain cancers, where increased permeability leads to inappropriate antigen exposure and tissue damage.
- Therapeutic Targets: Modulating tight junction integrity holds potential for enhancing drug delivery across epithelial barriers or restoring barrier function in disease states.
In summary, the structural components and organization of tight junctions are meticulously designed to create a selective barrier in epithelial tissues. Their ability to form tight seals, regulate permeability through diverse claudin interactions, and dynamically respond to cellular signals ensures the maintenance of distinct extracellular environments, cell polarity, and overall tissue homeostasis.
9. Explain the role of the sodium-potassium pump in maintaining the resting membrane potential and its significance in neuronal function.
Answer: The sodium-potassium pump (Na⁺/K⁺-ATPase) is a crucial membrane-bound enzyme that actively transports sodium (Na⁺) and potassium (K⁺) ions across the plasma membrane, playing a pivotal role in maintaining the resting membrane potential of cells, particularly neurons. Here’s a detailed explanation of its role and significance:
Mechanism of Action:
- Active Transport: The pump utilizes energy derived from the hydrolysis of ATP to transport ions against their concentration gradients. Specifically, it exports three Na⁺ ions out of the cell and imports two K⁺ ions into the cell per ATP molecule consumed.
- Phosphorylation Cycle: The pump undergoes conformational changes through a phosphorylation cycle. Binding of Na⁺ ions and ATP leads to phosphorylation, causing the pump to open towards the extracellular space and release Na⁺. Dephosphorylation then triggers a return to its original conformation, allowing K⁺ binding and subsequent transport into the cell.
Maintenance of Ion Gradients:
- Na⁺ Gradient: By continuously expelling Na⁺ ions, the pump maintains a low intracellular Na⁺ concentration compared to the extracellular environment. This gradient is essential for various cellular processes, including secondary active transport.
- K⁺ Gradient: Importing K⁺ ions keeps the intracellular concentration of K⁺ high relative to the outside. This gradient is vital for maintaining the resting membrane potential and for the repolarization phase of action potentials.
Contribution to Resting Membrane Potential:
- Electrochemical Balance: The selective permeability of the neuronal membrane, combined with the Na⁺ and K⁺ gradients established by the pump, creates an electrical gradient. K⁺ ions tend to diffuse out of the neuron, leaving behind negatively charged proteins, resulting in a negative resting membrane potential (~-70 mV).
- Sodium Leakage: Although Na⁺ ions tend to diffuse back into the cell passively due to the concentration gradient, the pump counteracts this by actively removing Na⁺, ensuring the maintenance of the negative potential.
- Energy Cost: The continuous activity of the Na⁺/K⁺-ATPase consumes a significant portion of the cell’s ATP, highlighting its importance in cellular energetics.
Significance in Neuronal Function:
- Action Potential Generation: The resting membrane potential established by the Na⁺/K⁺ pump is the baseline from which action potentials are generated. During an action potential, voltage-gated Na⁺ and K⁺ channels open, causing rapid depolarization and repolarization, respectively.
- Repolarization: After depolarization, the pump helps restore the original ion distribution, ensuring that neurons are ready to fire subsequent action potentials.
- Neurotransmitter Release: Proper ion gradients are essential for the maintenance of membrane potentials required for synaptic vesicle fusion and neurotransmitter release.
- Signal Propagation: The rapid and repetitive firing of action potentials in neurons relies on the efficient restoration of ion gradients by the Na⁺/K⁺ pump, enabling the propagation of electrical signals along axons.
- Cell Volume Regulation: By controlling ion concentrations, the pump indirectly regulates osmotic balance, preventing excessive swelling or shrinkage of neurons, which is crucial for maintaining their structural integrity.
Clinical Implications:
- Cardiac Glycosides: Drugs like digoxin inhibit the Na⁺/K⁺ pump, increasing intracellular Na⁺ levels, which indirectly raises intracellular Ca²⁺ via the Na⁺/Ca²⁺ exchanger. This enhances cardiac muscle contraction, useful in treating heart failure but potentially toxic if not properly managed.
- Neurological Disorders: Dysfunction of the Na⁺/K⁺ pump can lead to neurological symptoms, including seizures and muscle weakness, due to disrupted membrane potentials and impaired neuronal signaling.
- Genetic Mutations: Mutations in pump subunits can result in conditions such as familial hemiplegic migraine or alternating hemiplegia of childhood, characterized by disrupted ion homeostasis and neurological deficits.
In summary, the sodium-potassium pump is indispensable for establishing and maintaining the resting membrane potential in neurons. By actively regulating Na⁺ and K⁺ ion concentrations, it ensures the proper functioning of action potentials, neuronal communication, and overall brain activity. Its critical role underscores the importance of ion homeostasis in neuronal health and the potential consequences of pump dysfunction.
10. How do gap junctions facilitate intercellular communication, and what is their significance in synchronized cellular activities such as cardiac muscle contraction?
Answer: Gap junctions are specialized intercellular connections that form direct channels between adjacent cells, allowing the passage of ions, metabolites, and signaling molecules. They play a crucial role in facilitating intercellular communication and coordinating synchronized cellular activities. Here’s an in-depth look at their function and significance:
- Structure of Gap Junctions:
- Connexins: Gap junctions are composed of protein subunits called connexins. Six connexin molecules assemble to form a hemichannel or connexon on each cell surface.
- Formation of Channels: Two hemichannels from adjacent cells dock together to form a complete gap junction channel, establishing a continuous aqueous pathway between the cytoplasm of the connected cells.
- Permeability: The size and charge selectivity of gap junction channels allow the passage of molecules up to ~1 kDa in size, including ions, second messengers (e.g., cAMP, IP₃), and small metabolites.
- Mechanism of Intercellular Communication:
- Electrical Coupling: Gap junctions permit the direct flow of ions between cells, enabling electrical coupling. This is particularly important in excitable tissues where rapid and coordinated electrical signaling is essential.
- Chemical Coupling: They allow the transfer of signaling molecules and metabolic intermediates, facilitating biochemical communication and coordination of cellular responses.
- Synchronization: By enabling the equalization of ion concentrations and electrical potentials across connected cells, gap junctions synchronize cellular activities, ensuring cohesive tissue function.
- Significance in Synchronized Cellular Activities:
- Cardiac Muscle Contraction:
- Pacemaker Cells Coordination: In the heart, pacemaker cells generate spontaneous action potentials that propagate through gap junctions to neighboring cardiomyocytes, ensuring a unified heartbeat.
- Wave Propagation: The electrical signal spreads uniformly through the myocardium via gap junctions, allowing simultaneous contraction of cardiac muscle fibers, which is essential for effective blood pumping.
- Prevention of Arrhythmias: Proper gap junction function ensures regular and coordinated heart rhythms. Dysfunction or reduced expression of connexins can lead to arrhythmias and impaired cardiac function.
- Smooth Muscle and Peristalsis:
- Coordinated Contractions: In smooth muscle tissues, gap junctions enable the spread of electrical and chemical signals, coordinating contractions necessary for processes like peristalsis in the digestive tract.
- Neuronal Networks:
- Electrical Synapses: In certain parts of the nervous system, gap junctions form electrical synapses that allow rapid signal transmission between neurons, facilitating synchronized firing and network oscillations.
- Developmental Processes:
- Embryogenesis: Gap junctions enable communication between embryonic cells, coordinating differentiation and tissue patterning during development.
- Tissue Homeostasis:
- Metabolic Coordination: In tissues like the liver and kidneys, gap junctions allow the sharing of metabolic intermediates and regulatory molecules, maintaining overall tissue homeostasis.
- Cardiac Muscle Contraction:
- Regulation of Gap Junctions:
- Phosphorylation: Post-translational modifications of connexins can regulate gap junction assembly, disassembly, and permeability in response to cellular signals and environmental changes.
- pH and Ca²⁺ Sensitivity: Gap junction channels can be closed in response to high intracellular Ca²⁺ or acidic pH, protecting tissues from damage under stress conditions like ischemia.
- Clinical Implications:
- Heart Disease: Alterations in gap junction expression or function are linked to various cardiac diseases, including atrial fibrillation, ventricular tachycardia, and heart block.
- Cancer: Changes in gap junction communication can influence tumor growth and metastasis, as gap junctions can suppress uncontrolled cell proliferation.
- Inherited Disorders: Mutations in connexin genes (e.g., GJB2 encoding connexin 26) are associated with hearing loss and skin disorders, highlighting the importance of gap junctions in sensory and epithelial functions.
In summary, gap junctions are vital for direct intercellular communication, enabling the synchronized activity of cells within tissues. Their ability to allow the rapid exchange of ions and small molecules ensures coordinated physiological processes, such as the rhythmic contractions of the heart. Dysfunctional gap junctions can lead to significant health issues, underscoring their importance in maintaining synchronized and efficient cellular activities.
11. What is the significance of membrane fluidity in cellular processes, and how do cells regulate it under varying environmental conditions?
Answer: Membrane fluidity refers to the viscosity of the lipid bilayer of cell membranes, influencing the mobility of proteins and lipids within the membrane. It is critical for various cellular processes, including membrane protein function, vesicle formation, and cell signaling. Maintaining appropriate membrane fluidity is essential for cell survival and adaptability. Here’s a comprehensive look at its significance and regulatory mechanisms:
Significance of Membrane Fluidity:
- Protein Mobility and Function:
- Enzyme Activity: Fluid membranes allow enzymes and receptors to move freely, facilitating interactions necessary for catalysis and signal transduction.
- Signal Transduction: The lateral movement of receptors and associated signaling proteins within the fluid membrane is essential for effective signal propagation and amplification.
- Membrane Fusion and Vesicle Formation:
- Endocytosis and Exocytosis: Membrane fluidity enables the bending and fusion of membranes, critical for the formation of vesicles during endocytosis and the release of vesicles during exocytosis.
- Cell Movement and Shape Changes:
- Cytoskeletal Interactions: Fluid membranes accommodate the dynamic remodeling required for cell motility, shape changes, and the formation of structures like lamellipodia and filopodia.
- Lipid Rafts and Microdomain Formation:
- Organized Signaling Platforms: Membrane fluidity allows the transient clustering of specific lipids and proteins into lipid rafts, which serve as platforms for localized signaling events.
- Adaptation to Stress:
- Temperature Fluctuations: Fluid membranes enable cells to maintain functionality under varying temperatures by adjusting lipid composition to prevent rigidity or excessive fluidity.
- Membrane Integrity and Flexibility:
- Structural Stability: Adequate fluidity ensures that membranes remain intact and flexible, preventing rupture or excessive rigidity that can impede cellular functions.
- Protein Mobility and Function:
Regulation of Membrane Fluidity:
- Lipid Composition Adjustment:
- Unsaturated Fatty Acids: Incorporating unsaturated fatty acids with cis double bonds introduces kinks in the hydrocarbon chains, preventing tight packing and increasing fluidity.
- Saturated Fatty Acids: Increasing saturated fatty acids leads to tighter packing of lipids, reducing fluidity. Cells balance saturated and unsaturated fatty acids based on environmental needs.
- Cholesterol Content: Cholesterol modulates membrane fluidity by interacting with phospholipids. At high temperatures, cholesterol stabilizes the membrane and raises its melting point, reducing fluidity. At low temperatures, it prevents tight packing, increasing fluidity.
- Phospholipid Diversity:
- Variety of Head Groups: Different phospholipids with diverse head groups can influence membrane curvature and fluidity. For example, phosphatidylethanolamine induces negative curvature, while phosphatidylcholine promotes bilayer stability.
- Temperature Sensing and Response:
- Homeoviscous Adaptation: Cells adjust their lipid composition in response to temperature changes to maintain optimal membrane fluidity. For instance, bacteria incorporate branched-chain fatty acids at lower temperatures to prevent membrane stiffening.
- Sterol Regulation:
- Cholesterol Biosynthesis: Cells can upregulate or downregulate cholesterol synthesis to adjust membrane fluidity dynamically in response to environmental cues.
- Fatty Acid Desaturation:
- Desaturase Enzymes: These enzymes introduce double bonds into fatty acids, increasing the proportion of unsaturated fatty acids and enhancing membrane fluidity.
- Lipid Droplet Formation:
- Storage of Excess Lipids: Cells can sequester excess saturated fatty acids into lipid droplets, preventing their incorporation into the plasma membrane and maintaining fluidity.
- Environmental Stress Responses:
- Cold Shock Proteins: In some organisms, cold shock proteins can interact with membranes to stabilize fluidity under low-temperature conditions.
- Heat Shock Proteins: These proteins may help refold denatured membrane proteins and adjust lipid composition to prevent excessive fluidity at high temperatures.
- Lipid Composition Adjustment:
Impact of Dysregulated Membrane Fluidity:
- Protein Misfunction: Altered fluidity can impair the function of membrane proteins, affecting signaling, transport, and enzymatic activities.
- Increased Membrane Permeability: Excessive fluidity can lead to uncontrolled leakage of ions and molecules, disrupting cellular homeostasis.
- Cellular Stress and Death: Significant deviations from optimal fluidity can trigger stress responses, apoptosis, or necrosis, compromising cell viability.
In summary, membrane fluidity is a critical property that influences numerous cellular processes, from protein mobility and signal transduction to membrane fusion and cell movement. Cells meticulously regulate fluidity through adjustments in lipid composition, enzyme activity, and adaptive responses to environmental changes, ensuring that membrane functionality is maintained under diverse conditions. Proper regulation of membrane fluidity is essential for cellular integrity, adaptability, and overall physiological health.
12. How do cells utilize secondary active transport mechanisms to facilitate the movement of molecules against their concentration gradients, and what are some physiological examples of this process?
Answer: Secondary active transport mechanisms enable the movement of molecules against their concentration gradients by harnessing the energy stored in the electrochemical gradients of other ions, rather than directly using ATP. This process relies on the coupling of the transport of two different molecules: one moving down its gradient (providing energy) and the other moving against its gradient (receiving energy). There are two primary types of secondary active transport: symport (cotransport) and antiport (countertransport). Here’s how cells utilize these mechanisms and some physiological examples:
Mechanism of Secondary Active Transport:
- Symport (Cotransport): Both the driving ion and the transported molecule move in the same direction across the membrane. The movement of the driving ion down its gradient provides the energy to transport the second molecule against its gradient.
- Antiport (Countertransport): The driving ion and the transported molecule move in opposite directions. The movement of the driving ion down its gradient facilitates the transport of the second molecule against its gradient.
Physiological Examples:
Sodium-Glucose Transporter (SGLT):
- Type: Symport
- Location: Intestinal epithelial cells and renal proximal tubules
- Function: Couples the inward movement of Na⁺ ions down their electrochemical gradient with the simultaneous uptake of glucose against its concentration gradient. This mechanism is vital for glucose absorption from the diet and reabsorption in the kidneys.
- Clinical Relevance: SGLT inhibitors are used as therapeutic agents in the treatment of type 2 diabetes by preventing glucose reabsorption, thereby lowering blood glucose levels.
Sodium-Calcium Exchanger (NCX):
- Type: Antiport
- Location: Cardiac muscle cells and neurons
- Function: Utilizes the inward movement of Na⁺ ions to drive the outward transport of Ca²⁺ ions against their gradient. This is essential for terminating calcium signals and maintaining calcium homeostasis in cells.
- Physiological Importance: Critical for muscle relaxation in the heart and for resetting calcium levels after neuronal signaling.
Sodium-Potassium-Chloride Cotransporter (NKCC):
- Type: Symport
- Location: Renal cells, neurons, and secretory epithelia
- Function: Transports 1 Na⁺, 1 K⁺, and 2 Cl⁻ ions into the cell. This cotransport is important for maintaining ion balance, cell volume, and the osmotic gradient necessary for secondary active transport of other solutes.
- Physiological Role: In the kidneys, NKCC plays a key role in salt reabsorption and water balance.
Proton-Sucrose Symporter:
- Type: Symport
- Location: Plant cells
- Function: Couples the inward movement of H⁺ ions (protons) with the uptake of sucrose against its gradient. This is essential for sugar accumulation in plant cells, supporting energy storage and transport.
Na⁺/K⁺/2Cl⁻ Cotransporter (NKCC1):
- Type: Symport
- Location: Various tissues, including the kidney and neurons
- Function: Transports Na⁺, K⁺, and Cl⁻ ions into the cell, contributing to cell volume regulation and the maintenance of ionic gradients necessary for other transport processes.
Aspartate-Glutamate Transporter (EAAT):
- Type: Antiport
- Location: Neurons and glial cells
- Function: Couples the uptake of glutamate (a neurotransmitter) with the extrusion of Na⁺ and the influx of K⁺, facilitating the clearance of glutamate from the synaptic cleft and preventing excitotoxicity.
Sodium-Bicarbonate Cotransporter (NBC):
- Type: Symport
- Location: Renal tubules and astrocytes
- Function: Transports Na⁺ and HCO₃⁻ ions into the cell, aiding in pH regulation by buffering excess H⁺ ions and contributing to bicarbonate reabsorption in the kidneys.
Advantages of Secondary Active Transport:
- Energy Efficiency: Utilizes existing ion gradients established by primary active transport (e.g., Na⁺/K⁺ pump), conserving cellular ATP.
- Coupled Transport: Allows cells to coordinate the movement of multiple ions and solutes, integrating various physiological processes.
- Versatility: Supports the uptake and extrusion of a wide range of molecules, including sugars, amino acids, ions, and neurotransmitters.
Regulation of Secondary Active Transporters:
- Expression Levels: Cells can modulate the number of transporters on the membrane to adjust uptake or extrusion rates based on physiological needs.
- Post-Translational Modifications: Phosphorylation and other modifications can alter transporter activity, influencing their affinity and transport rates.
- Feedback Mechanisms: Changes in intracellular ion concentrations or signaling pathways can regulate transporter function, ensuring tight control over molecular movement.
In summary, secondary active transport mechanisms are vital for the selective and efficient movement of molecules against their concentration gradients, leveraging existing ion gradients to drive essential physiological processes. Examples such as glucose uptake in the intestines, calcium extrusion in neurons, and ion balance in renal cells illustrate the fundamental roles these transporters play in maintaining cellular and organismal homeostasis.