Cell communication is a fundamental process that enables living organisms to maintain internal coordination, respond to external cues, and function as integrated systems. This intricate signaling network governs everything from growth and immunity to behavior and development. As a critical topic in science, the study of cell communication builds on foundational knowledge from broader fields such as biology and cell biology, revealing the molecular mechanisms through which cells detect, transmit, and respond to signals.
At the core of this subject lies the understanding of how cells use receptors, signaling molecules, and cascades to achieve specific outcomes. The structure of a cell itself, explored in cell structure, provides the architecture for receiving and transmitting messages. These messages may influence vital processes such as the cell cycle, cell development, and cell physiology, which are all under tight regulation by signal transduction pathways.
Beyond individual cells, communication plays a central role in multicellular organization and environmental interactions. For example, signaling mechanisms are essential in ecological contexts such as predator-prey responses or interspecies symbiosis, as studied in ecology. On evolutionary scales, the refinement of communication networks has contributed to organismal complexity and specialization, as shown in evolutionary biology.
Cell communication is also a gateway to understanding genetic regulation. In genetics and its subfields, communication underlies gene regulation mechanisms such as gene expression and protein synthesis. These processes are dependent on molecular signals that activate or suppress specific genes. A thorough understanding of molecular genetics illuminates how DNA and RNA act as intermediaries between communication signals and functional outputs.
Mutations and errors in signaling often lead to profound consequences, such as those explored in genetic mutation and inheritance. These disruptions provide insight into hereditary diseases, cancer, and developmental disorders. Research into applications of genetics in medicines relies heavily on understanding cell signaling pathways, many of which are targeted in drug design and precision therapies.
As science progresses, advanced technologies continue to expand our knowledge of communication at the molecular level. Tools such as DNA technology and molecular techniques in research enable scientists to dissect complex interactions with unprecedented accuracy. These advances feed into large-scale genomic studies, including genomics, and support the exploration of evolutionary adaptations through molecular evolution.
From classical models like Mendelian genetics to population-level analyses in population genetics and quantitative genetics, the ability of cells to communicate underpins all life processes. As students navigate this topic, they gain essential insight into the inner workings of cells, the causes of disease, and the future of biological innovation.
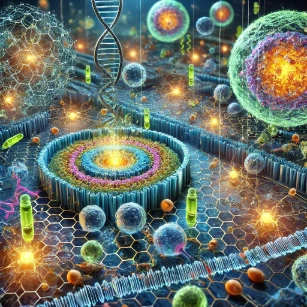
Table of Contents
Cell Communication and Signaling
Cell communication and signaling are fundamental processes that allow cells to sense, respond, and adapt to changes in their environment and coordinate activities with other cells. This communication ensures that multicellular organisms can function as integrated systems, regulating growth, development, metabolism, and immune responses. Understanding these pathways is crucial in fields such as immunology, neurobiology, and cancer research, as disruptions in signaling mechanisms often underlie diseases.
Overview of Cell Communication
Cell communication involves signaling pathways that transmit information from the external environment or neighboring cells to trigger a specific cellular response. This process occurs in three main stages:
- Reception: A signaling molecule (ligand) binds to a specific receptor on the cell surface or inside the cell.
- Transduction: The signal is relayed through a series of intracellular molecules, often involving second messengers or protein phosphorylation cascades.
- Response: The cell produces a specific response, such as activating a gene, altering metabolism, or changing cell behavior.
Signal Transduction Pathways
Signal transduction pathways act as molecular circuits that relay and amplify signals received by the cell. These pathways involve a precise sequence of molecular interactions that ensure specificity and efficiency in the cellular response.
a. Key Signaling Molecules
- Hormones: Chemical messengers like insulin, epinephrine, and growth hormones that regulate metabolism, stress responses, and development.
- Growth Factors: Proteins such as epidermal growth factor (EGF) and platelet-derived growth factor (PDGF) that stimulate cell division and tissue regeneration.
- Neurotransmitters: Molecules like dopamine, acetylcholine, and serotonin that transmit signals across synapses in the nervous system.
b. Steps in Signal Transduction
- Ligand Binding: A ligand binds to its receptor, inducing a conformational change that activates the receptor.
- Signal Relay: Intracellular proteins such as G-proteins, kinases, and second messengers like cAMP or calcium ions propagate the signal.
- Amplification: Each step may activate multiple downstream targets, resulting in amplification of the original signal.
- Response: The cell may respond in various ways, including:
- Activating or inhibiting enzymes to modify cellular metabolism.
- Initiating transcription factors that alter gene expression.
- Triggering structural changes such as cytoskeletal reorganization or cell motility.
For an interactive tutorial on how signal transduction pathways work in different organisms, you can explore the Nature Scitable resource on cell signaling.
Cell Membrane Receptors
Cell membrane receptors serve as essential gatekeepers, detecting extracellular signals and translating them into intracellular actions. These proteins are embedded in the lipid bilayer and are specifically tuned to recognize hormones, neurotransmitters, growth factors, and other chemical messengers. Their ability to initiate complex signaling cascades makes them vital to regulating physiological processes such as metabolism, immune response, neural activity, and cellular growth.
a. G-Protein Coupled Receptors (GPCRs)
- Structure: GPCRs are a large family of receptors characterized by their seven transmembrane alpha-helical domains. These receptors interact with heterotrimeric G-proteins located on the cytoplasmic side of the membrane.
- Mechanism:
- A ligand, such as a hormone or neurotransmitter, binds to the extracellular portion of the GPCR.
- This binding causes a conformational change in the receptor, activating the associated G-protein by exchanging GDP for GTP on the alpha subunit.
- The activated G-protein then modulates the activity of target enzymes like adenylyl cyclase (which generates cyclic AMP) or phospholipase C (which produces IP₃ and DAG), leading to the production of second messengers such as cAMP or Ca²⁺.
- Example: The binding of epinephrine to beta-adrenergic receptors in liver cells activates the cAMP signaling pathway, ultimately resulting in glycogen breakdown and increased glucose availability.
b. Ion Channel-Linked Receptors
- Function: Also known as ligand-gated ion channels, these receptors open or close in response to the binding of a specific ligand. This rapid response allows the passage of ions such as Na⁺, K⁺, Ca²⁺, or Cl⁻ into or out of the cell, directly altering membrane potential and cellular excitability.
- Example: In neuromuscular junctions, acetylcholine binds to nicotinic acetylcholine receptors, opening sodium channels. The resulting influx of Na⁺ depolarizes the muscle membrane, initiating contraction.
c. Enzyme-Linked Receptors
- Function: These receptors either have intrinsic enzymatic activity or are directly associated with enzymes. Upon ligand binding, the receptor’s cytoplasmic domain initiates enzymatic reactions, frequently involving phosphorylation of target proteins.
- Example: Receptor tyrosine kinases (RTKs) represent the most prominent class of enzyme-linked receptors. When insulin binds to its RTK, it triggers a cascade that leads to glucose uptake by recruiting glucose transporters to the membrane.
- This process is crucial for maintaining blood sugar homeostasis and is the basis for many therapeutic interventions in diabetes.
Membrane receptors are key drug targets, with over one-third of all pharmaceuticals acting on them. For more detailed insights into how receptor-ligand interactions shape cellular responses and therapeutic design, refer to the NCBI resource on cell signaling pathways.
Mechanisms of Cell-to-Cell Communication
Cells employ a diverse range of communication mechanisms to coordinate behavior, regulate development, maintain homeostasis, and respond to environmental changes. The method of communication depends on the spatial relationship between the signaling and target cells, as well as the nature of the signal molecules involved. Understanding these mechanisms is essential for fields ranging from immunology to developmental biology and cancer research.
a. Direct Contact
- Gap Junctions: These are specialized intercellular connections formed by protein complexes called connexins, which create aqueous channels that permit the direct exchange of ions, metabolites, and signaling molecules between adjacent cells.
- Example: In cardiac muscle tissue, gap junctions synchronize the contraction of heart cells, ensuring a unified and rhythmic heartbeat.
- Cell-Cell Recognition: This occurs when membrane-bound surface molecules on one cell interact with receptors on another cell. Such recognition events are crucial for immune responses, tissue formation, and cellular differentiation.
- Example: T cells in the immune system identify infected or abnormal cells by binding to specific antigens presented on their surface via major histocompatibility complex (MHC) proteins.
b. Paracrine Signaling
- Definition: In paracrine communication, signaling molecules are secreted by a cell and influence neighboring target cells within a short distance. These molecules usually have rapid effects and are quickly degraded or taken up to restrict their range of action.
- Example: Fibroblast Growth Factor (FGF) is a classic paracrine signal that promotes the proliferation and differentiation of nearby cells during embryonic development, wound healing, and tissue repair.
c. Synaptic Signaling
- Definition: A specialized form of paracrine signaling observed in the nervous system, where neurotransmitters are released by neurons into synaptic clefts to transmit signals to adjacent neurons, muscle cells, or glands.
- Example: The neurotransmitter acetylcholine is released by motor neurons and binds to receptors on skeletal muscle cells, initiating contraction and motor responses.
d. Endocrine Signaling
- Definition: In endocrine signaling, hormones are released into the bloodstream by endocrine glands and travel throughout the body to regulate distant target cells. This mechanism enables long-range coordination of physiological functions.
- Example: Insulin, secreted by the beta cells of the pancreas, circulates through the bloodstream to regulate glucose uptake and metabolism in muscle and liver cells.
e. Autocrine Signaling
- Definition: In autocrine signaling, a cell produces signaling molecules that bind to receptors on its own surface, creating a self-stimulating feedback loop. This type of communication plays a role in development and immune regulation, and is often dysregulated in cancer.
- Example: Tumor cells often exploit autocrine loops by releasing growth factors such as TGF-α or VEGF, which in turn bind to their own receptors to promote unchecked proliferation and angiogenesis.
Cell-to-cell communication is critical to life and is a central focus of biomedical science. Emerging techniques like single-cell transcriptomics and optogenetics are offering unprecedented insights into these communication pathways. For a broader overview, the Khan Academy cell signaling guide provides accessible explanations and examples for learners at all levels.
Role of Cell Signaling in Key Biological Processes
Cell signaling is integral to the coordination and regulation of numerous physiological processes in multicellular organisms. By transmitting information both within and between cells, signaling pathways orchestrate critical functions such as development, immunity, metabolism, and neural activity. Disruptions in these pathways can result in diseases, including cancer, autoimmune disorders, and neurological conditions.
a. Cell Growth and Division
- Growth factors such as Epidermal Growth Factor (EGF) bind to receptor tyrosine kinases (RTKs) on the cell membrane, initiating a phosphorylation cascade that activates transcription factors and drives the expression of genes involved in cell cycle progression.
- This process ensures that cells divide only when necessary—for example, during development or tissue repair.
- Dysregulation: Mutations in growth factor receptors or downstream signaling components, like RAS or MAPK, can lead to uncontrolled proliferation, a hallmark of many cancers. Targeted therapies often aim to inhibit these overactive pathways.
b. Immune Response
- Effective immune defense relies heavily on precise cell signaling between immune cells. Cytokines (e.g., interleukins, interferons) and chemokines serve as messengers that activate, attract, or regulate immune cells during infections and inflammation.
- T-cells recognize foreign antigens presented by infected or abnormal cells using their T-cell receptors (TCRs). Upon activation, they release signaling molecules such as interleukin-2 (IL-2) to recruit and coordinate helper T cells, cytotoxic T cells, and B cells.
- Errors in immune signaling can result in immunodeficiency or autoimmunity, highlighting the need for finely tuned communication networks.
c. Nervous System Communication
- The nervous system depends on chemical signaling at synapses to transmit information rapidly and specifically between neurons or between neurons and muscles or glands.
- Neurotransmitters such as dopamine, serotonin, acetylcholine, and glutamate are released into the synaptic cleft and bind to receptors on the postsynaptic membrane, triggering electrical or metabolic responses.
- Dopamine, in particular, plays crucial roles in mood regulation, movement coordination, and reward processing. Disruption of dopamine signaling is implicated in disorders like Parkinson’s disease and schizophrenia.
- For a foundational review of neurotransmitter function and receptor types, the NCBI Neuroscience Textbook provides comprehensive educational resources.
d. Metabolic Regulation
- Hormones are central to metabolic control, with insulin and glucagon maintaining blood glucose homeostasis. These hormones act on receptors located on the surface of liver, muscle, and adipose cells to modulate nutrient uptake and storage.
- Insulin signaling activates the PI3K/AKT pathway, leading to the translocation of GLUT4 transporters to the cell membrane, thereby enhancing glucose uptake into the cell.
- Conversely, glucagon signaling in hepatocytes triggers glycogenolysis and gluconeogenesis, ensuring adequate glucose levels during fasting states.
- Defects in insulin signaling are at the core of metabolic diseases such as Type 2 diabetes, making it a prime target for therapeutic intervention.
Cell signaling is thus a central theme in modern biology and medicine, linking molecular events to whole-organism physiology. It serves as a unifying principle for understanding health, disease, and the therapeutic mechanisms that restore biological balance.
Disorders Associated with Defective Cell Signaling
Precise and regulated cell signaling is essential for maintaining health in multicellular organisms. When signaling pathways malfunction due to genetic mutations, environmental factors, or cellular stress, they can give rise to a range of disorders. These diseases often stem from either excessive signaling, insufficient signaling, or signaling in the wrong context. Below are some major disorders linked to dysfunctional cell communication mechanisms:
- Cancer: One of the most well-documented consequences of aberrant cell signaling is cancer. Mutations in genes encoding receptor tyrosine kinases (RTKs), such as HER2 or EGFR, or in downstream proteins like RAS, BRAF, or PI3K, can cause cells to divide uncontrollably even in the absence of external growth cues. These pathways are often constitutively activated, bypassing normal cell cycle checkpoints and promoting tumor formation, metastasis, and resistance to apoptosis. Targeted therapies like tyrosine kinase inhibitors (e.g., erlotinib, imatinib) have emerged from understanding these signaling defects.
- Diabetes: This metabolic disorder results primarily from impaired insulin signaling. In Type 2 diabetes, insulin receptors on muscle and adipose tissues become resistant to insulin’s action, disrupting the PI3K-AKT pathway and reducing glucose uptake. This causes elevated blood glucose levels, which, over time, damage blood vessels, nerves, and organs. Research published by the American Diabetes Association emphasizes the critical role of restoring signaling sensitivity in treatment strategies.
- Neurodegenerative Diseases: Proper neuronal communication via neurotransmitters is vital for brain function. In diseases like Parkinson’s, a reduction in dopamine-producing neurons impairs movement coordination and reward signaling. Alzheimer’s disease is linked to disrupted synaptic signaling, often due to the accumulation of amyloid-beta plaques and tau tangles that interfere with neuronal receptors and synaptic transmission. Restoring or preserving neurotransmission is a major therapeutic goal.
- Autoimmune Diseases: The immune system relies on highly regulated signaling to distinguish self from non-self. In autoimmune conditions such as multiple sclerosis, rheumatoid arthritis, or lupus, signaling errors lead to the misidentification of healthy tissues as threats. This results in chronic inflammation and tissue destruction. Defective cytokine signaling and failure in T-cell receptor (TCR) tolerance checkpoints are commonly implicated. Modulating immune signaling with biologics like TNF inhibitors or IL-6 blockers has shown success in managing these disorders.
These examples underscore how essential signaling fidelity is to cellular health. By unraveling the molecular underpinnings of defective communication, researchers can develop targeted therapies that correct or bypass faulty signaling components, offering more precise and effective treatments.
Why Study Cell Communication
Coordination of Biological Processes
Cell communication ensures that cells can coordinate activities such as growth, immune response, and tissue repair. This communication is vital for maintaining homeostasis within multicellular organisms. Understanding these signaling pathways helps explain how cells work together as a system.
Key to Understanding Diseases
Many diseases arise from faulty cell signaling, including autoimmune disorders, diabetes, and cancers. Studying how cells communicate can reveal the root causes of these conditions. This knowledge aids in developing targeted therapies and medical interventions.
Insight into Drug Mechanisms
Pharmaceuticals often target specific cell signaling pathways to treat diseases. By understanding these pathways, students can appreciate how drugs exert their effects. This is essential for careers in pharmacology, medicine, and biomedical engineering.
Impact on Development and Differentiation
Cell communication influences how cells differentiate and form specialized tissues during development. This is crucial for understanding embryology and regenerative medicine. Knowledge in this area supports innovations in tissue engineering and stem cell therapy.
Relevance in Environmental and Microbial Interactions
Cells communicate not only within organisms but also with their environment and other species, such as bacteria. This intercellular signaling affects ecosystems, immunity, and host-pathogen interactions. A deeper understanding enhances research in ecology, microbiology, and public health.
Cell Communication and Signaling: Conclusion
Cell communication and signaling lie at the heart of biological coordination and regulation. These intricate processes enable cells to perceive changes in their environment, interact with neighboring cells, and carry out synchronized responses essential for survival and function. From embryonic development and tissue repair to immune defense and neural activity, signal transduction pathways govern nearly every aspect of life in multicellular organisms.
The study of receptors, second messengers, and downstream effectors has uncovered how finely tuned molecular conversations guide cell fate, shape tissue dynamics, and influence systemic responses. Importantly, disruptions in signaling can trigger serious health issues, including uncontrolled cell growth in cancer, metabolic imbalances in diabetes, and cognitive decline in neurodegenerative diseases. Therapeutic breakthroughs—from targeted antibodies and kinase inhibitors to hormone replacement and neurotransmitter modulation—are direct outcomes of our growing understanding of these pathways.
Moreover, advances in cell signaling research continue to drive innovation in regenerative medicine, immunotherapy, and personalized healthcare. As we decode more signaling networks, we not only enhance our capacity to treat disease but also deepen our insight into the remarkable complexity and adaptability of living systems.
Cell Communication and Signaling: Review Questions with Detailed Answers
Q1. What are the different types of cell signaling, and how do they differ in terms of range and target specificity?
Answer:
Types of Cell Signaling:
Autocrine Signaling:
- Definition: Cells respond to signals they themselves produce.
- Range: Limited to the producing cell.
- Target Specificity: High specificity as the signal affects the same cell that secretes it.
- Example: T cells in the immune system release cytokines that act on themselves to promote their own activation and proliferation.
Paracrine Signaling:
- Definition: Signals are released by one cell and affect nearby target cells.
- Range: Short-distance communication within a tissue.
- Target Specificity: Moderate specificity, affecting multiple nearby cells.
- Example: Neurotransmitters released by neurons act on adjacent neurons or muscle cells.
Endocrine Signaling:
- Definition: Signals (hormones) are released into the bloodstream to reach distant target cells.
- Range: Long-distance communication across the entire body.
- Target Specificity: Low specificity, as hormones can potentially affect multiple organs and tissues.
- Example: Insulin secreted by the pancreas regulates glucose uptake in various cells throughout the body.
Juxtacrine Signaling (Contact-Dependent Signaling):
- Definition: Signals require direct contact between the signaling and target cells, often involving membrane-bound ligands and receptors.
- Range: Immediate, as cells must be in direct contact.
- Target Specificity: High specificity due to direct cell-cell interaction.
- Example: Notch signaling pathway, where the Notch receptor on one cell interacts with its ligand on an adjacent cell to influence cell fate decisions.
Differences in Range and Target Specificity:
Range:
- Autocrine and Juxtacrine: Short-range, affecting the same or adjacent cells.
- Paracrine: Local, affecting nearby cells within the same tissue.
- Endocrine: Systemic, affecting cells throughout the body via the bloodstream.
Target Specificity:
- High Specificity: Autocrine and Juxtacrine signaling due to direct or self-targeting interactions.
- Moderate Specificity: Paracrine signaling as signals affect multiple nearby cells.
- Low Specificity: Endocrine signaling since hormones can influence a wide array of cell types and tissues.
Conclusion: Understanding the different types of cell signaling is crucial for comprehending how cells communicate and coordinate functions within tissues and the entire organism. Each signaling type serves distinct roles in maintaining physiological balance, responding to environmental changes, and regulating development and immune responses.
Q2. Describe the role of receptors in cell signaling and differentiate between G-protein coupled receptors (GPCRs) and receptor tyrosine kinases (RTKs).
Answer:
Role of Receptors in Cell Signaling: Receptors are specialized proteins located on the cell surface or within the cell that detect and bind to signaling molecules (ligands). Upon ligand binding, receptors undergo conformational changes that initiate intracellular signal transduction pathways, leading to specific cellular responses such as gene expression, metabolism regulation, or cell movement.
Types of Receptors:
G-Protein Coupled Receptors (GPCRs):
- Structure: Seven transmembrane α-helices connected by extracellular and intracellular loops. Associated with heterotrimeric G-proteins (composed of α, β, and γ subunits).
- Mechanism:
- Ligand Binding: The extracellular domain binds to a ligand (e.g., hormones, neurotransmitters).
- Conformational Change: Induces a change in the receptor’s structure, activating the associated G-protein by facilitating the exchange of GDP for GTP on the α subunit.
- Signal Transduction: The activated G-protein dissociates into Gα and Gβγ subunits, which then interact with downstream effectors (e.g., adenylate cyclase, phospholipase C) to generate secondary messengers (e.g., cAMP, IP₃, DAG).
- Termination: GTP is hydrolyzed to GDP, reassembling the inactive G-protein.
- Examples: Adrenergic receptors, muscarinic acetylcholine receptors.
Receptor Tyrosine Kinases (RTKs):
- Structure: Single-pass transmembrane proteins with an extracellular ligand-binding domain, a single transmembrane helix, and an intracellular tyrosine kinase domain.
- Mechanism:
- Ligand Binding: The extracellular domain binds to a specific ligand (e.g., growth factors).
- Dimerization: Ligand binding induces receptor dimerization (pairing of two RTK molecules).
- Autophosphorylation: The intracellular tyrosine kinase domains phosphorylate each other on specific tyrosine residues.
- Signal Transduction: Phosphorylated tyrosines serve as docking sites for downstream signaling proteins containing SH2 (Src Homology 2) domains, activating pathways such as MAPK, PI3K/Akt, and PLCγ.
- Termination: Phosphatases remove phosphate groups, inactivating the receptor.
- Examples: Epidermal Growth Factor Receptor (EGFR), Insulin Receptor.
Differentiation Between GPCRs and RTKs:
Feature | GPCRs | RTKs |
---|---|---|
Structure | Seven transmembrane α-helices | Single-pass transmembrane with tyrosine kinase domain |
Ligand Types | Small molecules (hormones, neurotransmitters) | Large proteins (growth factors, hormones) |
Signal Transduction | G-protein mediated, generating secondary messengers | Direct phosphorylation cascades, recruiting adaptor proteins |
Activation | Ligand-induced conformational change activating G-proteins | Ligand-induced dimerization and autophosphorylation |
Secondary Messengers | cAMP, IP₃, DAG, Ca²⁺ | Mainly protein phosphorylation cascades |
Examples | Beta-adrenergic receptors, rhodopsin | EGFR, Insulin receptor |
Functional Roles | Diverse roles including sensory perception, neurotransmission | Primarily involved in growth, differentiation, metabolism |
Conclusion: GPCRs and RTKs are critical receptors in cell signaling, each with distinct structures and mechanisms of action. GPCRs are versatile receptors that interact with a wide range of ligands and utilize G-proteins to generate diverse secondary messengers. In contrast, RTKs primarily respond to growth factors and initiate specific phosphorylation cascades that regulate cell growth, differentiation, and metabolism. Understanding these receptors is fundamental to comprehending cellular communication and the development of targeted therapies for various diseases.
Q3. Explain the concept of signal transduction and outline the general steps involved in a typical signal transduction pathway.
Answer:
Concept of Signal Transduction: Signal transduction refers to the series of molecular events by which a cell converts an extracellular signal (ligand binding) into a specific intracellular response. This process involves the transmission and amplification of signals through various biochemical pathways, ultimately leading to changes in gene expression, enzyme activity, or cellular behavior.
General Steps in a Typical Signal Transduction Pathway:
Signal Reception:
- Ligand Binding: A signaling molecule (ligand) binds to a specific receptor protein located on the cell surface or within the cell.
- Receptor Activation: Ligand binding induces a conformational change in the receptor, activating its intrinsic activity or facilitating the recruitment of downstream signaling molecules.
Signal Transduction:
- Relay Mechanisms: Activated receptors initiate a cascade of intracellular events, often involving phosphorylation, G-proteins, or secondary messengers.
- Amplification: A single ligand-receptor interaction can activate multiple downstream molecules, amplifying the signal within the cell.
- Integration: Signals from multiple pathways can converge, allowing the cell to integrate diverse information and coordinate complex responses.
Signal Amplification:
- Secondary Messengers: Small molecules (e.g., cAMP, IP₃, DAG, Ca²⁺) are generated or released to propagate the signal further into the cell.
- Cascade Amplification: Sequential activation of enzymes (e.g., kinases) leads to a rapid and substantial amplification of the initial signal.
Response Generation:
- Gene Expression: Transcription factors are activated to modulate the expression of specific genes, resulting in the synthesis of proteins that execute the cellular response.
- Enzyme Activation/Inhibition: Direct modification of enzyme activities alters metabolic pathways and cellular functions.
- Cytoskeletal Rearrangement: Changes in the cytoskeleton facilitate cellular movements or shape changes.
- Apoptosis: Initiation of programmed cell death in response to specific signals.
Signal Termination:
- Deactivation of Receptors: Ligands are removed or degraded, and receptors return to their inactive state.
- Degradation of Secondary Messengers: Enzymes break down secondary messengers to stop signal propagation.
- Feedback Inhibition: Activated signaling components inhibit earlier steps in the pathway to prevent overactivation.
Example of a Signal Transduction Pathway: The cAMP Pathway
- Signal Reception:
- Ligand: Epinephrine binds to a GPCR on the cell membrane.
- Signal Transduction:
- GPCR Activation: Conformational change activates the associated G-protein by exchanging GDP for GTP.
- Signal Amplification:
- Adenylyl Cyclase Activation: Gα subunit activates adenylyl cyclase, converting ATP to cAMP.
- Multiple cAMP Molecules: Each activated adenylyl cyclase produces multiple cAMP molecules, amplifying the signal.
- Response Generation:
- Protein Kinase A (PKA) Activation: cAMP binds to the regulatory subunits of PKA, releasing and activating the catalytic subunits.
- Phosphorylation of Targets: Activated PKA phosphorylates various proteins, leading to metabolic changes such as glycogen breakdown.
- Signal Termination:
- Phosphodiesterase Activity: Enzyme breaks down cAMP into AMP, reducing PKA activity.
- GTP Hydrolysis: Gα subunit hydrolyzes GTP to GDP, inactivating the G-protein.
Conclusion: Signal transduction is a fundamental process that enables cells to perceive and respond to their environment. By understanding the general steps and specific pathways involved, researchers can elucidate how cells communicate, make decisions, and maintain homeostasis. Disruptions in signal transduction pathways are often linked to diseases, making them critical targets for therapeutic interventions.
Q4. Discuss the role of second messengers in signal transduction pathways and provide examples of common second messengers and their functions.
Answer:
Role of Second Messengers: Second messengers are small, non-protein molecules that transmit signals from receptors on the cell surface to target molecules inside the cell. They amplify and propagate the signal initiated by the binding of a first messenger (e.g., hormones, neurotransmitters) to its receptor, enabling a rapid and coordinated cellular response.
Functions of Second Messengers:
Signal Amplification:
- A single activated receptor can generate multiple second messenger molecules, amplifying the initial signal and ensuring a robust response.
Signal Specificity:
- Different second messengers are involved in distinct signaling pathways, ensuring that specific cellular responses are elicited based on the signal received.
Spatial and Temporal Regulation:
- Second messengers can rapidly diffuse within the cell, allowing signals to reach various intracellular targets efficiently and in a timely manner.
Common Second Messengers and Their Functions:
Cyclic Adenosine Monophosphate (cAMP):
- Function: Activates Protein Kinase A (PKA), which phosphorylates target proteins to regulate metabolism, gene expression, and other cellular processes.
- Example: In the liver, cAMP-PKA pathway promotes glycogen breakdown in response to epinephrine.
Inositol 1,4,5-Triphosphate (IP₃) and Diacylglycerol (DAG):
- Function:
- IP₃: Triggers the release of Ca²⁺ from the endoplasmic reticulum, increasing intracellular calcium levels.
- DAG: Activates Protein Kinase C (PKC), which phosphorylates various target proteins involved in cell growth, differentiation, and apoptosis.
- Example: Activation of phospholipase C by RTKs leads to the production of IP₃ and DAG, initiating calcium signaling and PKC activation.
- Function:
Calcium Ions (Ca²⁺):
- Function: Acts as a versatile second messenger regulating processes such as muscle contraction, neurotransmitter release, enzyme activity, and gene expression.
- Example: In muscle cells, increased Ca²⁺ levels trigger the interaction of actin and myosin for contraction.
Cyclic Guanosine Monophosphate (cGMP):
- Function: Activates Protein Kinase G (PKG), involved in processes like vasodilation, phototransduction in the retina, and smooth muscle relaxation.
- Example: Nitric oxide (NO) stimulates the production of cGMP, leading to the relaxation of vascular smooth muscle and vasodilation.
Nitric Oxide (NO):
- Function: Diffuses freely across membranes to activate guanylate cyclase, increasing cGMP levels and promoting vasodilation and neurotransmission.
- Example: NO release in endothelial cells causes relaxation of adjacent smooth muscle cells, lowering blood pressure.
Phosphatidylinositol (3,4,5)-Triphosphate (PIP₃):
- Function: Activates Akt/PKB, a key regulator of cell survival, growth, and metabolism.
- Example: Growth factor signaling leads to PIP₃ production, promoting cell proliferation and survival through Akt activation.
Examples of Second Messenger Pathways:
cAMP Pathway:
- Ligand: Epinephrine binds to β-adrenergic GPCR.
- Second Messenger: cAMP activates PKA.
- Response: PKA phosphorylates enzymes involved in glycogen breakdown.
IP₃/DAG Pathway:
- Ligand: Angiotensin II binds to AT1 receptor (a GPCR).
- Second Messengers: IP₃ induces Ca²⁺ release; DAG activates PKC.
- Response: PKC phosphorylates proteins regulating vascular smooth muscle contraction, increasing blood pressure.
Conclusion: Second messengers are essential components of signal transduction pathways, enabling the amplification and precise regulation of cellular responses to external signals. By understanding the roles and mechanisms of common second messengers, researchers can elucidate how cells coordinate complex physiological processes and respond to environmental changes. Dysregulation of second messenger systems is often implicated in various diseases, highlighting their importance in cellular function and health.
Q5. What are the main steps involved in the G-protein coupled receptor (GPCR) signaling pathway, and how does this pathway lead to cellular responses?
Answer:
G-Protein Coupled Receptor (GPCR) Signaling Pathway Overview: GPCRs are a large family of membrane receptors that respond to a diverse array of extracellular signals, including hormones, neurotransmitters, and sensory stimuli. Upon ligand binding, GPCRs activate heterotrimeric G-proteins, which then initiate various intracellular signaling cascades leading to specific cellular responses.
Main Steps in the GPCR Signaling Pathway:
Ligand Binding:
- Activation: An extracellular signaling molecule (ligand) binds to the extracellular domain of the GPCR, inducing a conformational change in the receptor.
G-Protein Activation:
- GDP-GTP Exchange: The conformational change in the GPCR facilitates the exchange of GDP for GTP on the α subunit of the associated heterotrimeric G-protein (composed of α, β, and γ subunits).
- Dissociation: Binding of GTP causes the Gα subunit to dissociate from the Gβγ dimer, allowing both to interact with downstream effectors independently.
Effector Activation:
- Gα Subunit:
- Type-Specific Actions: Depending on the type of Gα subunit (Gs, Gi, Gq, etc.), different effectors are activated.
- Gs (Stimulatory): Activates adenylyl cyclase to increase cAMP levels.
- Gi (Inhibitory): Inhibits adenylyl cyclase, decreasing cAMP levels.
- Gq: Activates phospholipase C (PLC), leading to the production of IP₃ and DAG.
- Type-Specific Actions: Depending on the type of Gα subunit (Gs, Gi, Gq, etc.), different effectors are activated.
- Gβγ Dimer:
- Direct Effector Interaction: Can directly activate or inhibit various enzymes and ion channels, such as ion channels or PI3K.
- Gα Subunit:
Second Messenger Generation:
- cAMP Pathway (Gs): Activation of adenylyl cyclase converts ATP to cAMP, which activates Protein Kinase A (PKA).
- IP₃/DAG Pathway (Gq): PLC cleaves PIP₂ into IP₃ and DAG. IP₃ releases Ca²⁺ from the endoplasmic reticulum, and DAG activates Protein Kinase C (PKC).
- Others: Different Gα subunits can activate other second messengers like nitric oxide (NO) or cyclic GMP (cGMP).
Signal Amplification:
- Multiple Targets: Each second messenger can activate multiple downstream targets, amplifying the initial signal.
- Kinase Cascades: Activation of kinases leads to phosphorylation of numerous target proteins, further propagating the signal.
Cellular Response:
- Gene Expression: Activated kinases can phosphorylate transcription factors, altering gene expression.
- Metabolic Changes: Enzymes involved in metabolism can be activated or inhibited, leading to changes in cellular metabolic activities.
- Ion Channel Regulation: Modulation of ion channels affects cellular excitability, muscle contraction, or neurotransmitter release.
- Cytoskeletal Reorganization: Changes in the cytoskeleton influence cell shape, movement, and division.
Signal Termination:
- GTP Hydrolysis: The intrinsic GTPase activity of the Gα subunit hydrolyzes GTP to GDP, terminating the signal.
- Reassociation: Gα-GDP reassociates with the Gβγ dimer, reforming the inactive heterotrimeric G-protein.
- Receptor Desensitization: Receptors can be phosphorylated by GRKs (GPCR kinases) and bind to arrestins, preventing further G-protein activation.
Example: β-Adrenergic Receptor Signaling (Gs Pathway):
- Ligand Binding: Epinephrine binds to the β-adrenergic GPCR on the cell membrane.
- G-Protein Activation: GPCR activates Gs protein, causing Gαs to bind GTP and dissociate from Gβγ.
- Effector Activation: Gαs activates adenylyl cyclase.
- Second Messenger Generation: Adenylyl cyclase converts ATP to cAMP.
- Signal Amplification: cAMP activates multiple PKA molecules.
- Cellular Response: PKA phosphorylates target proteins, leading to increased glycogen breakdown and glucose release into the bloodstream.
- Signal Termination: GTP on Gαs is hydrolyzed to GDP, inactivating Gαs and terminating the signal.
Conclusion: The GPCR signaling pathway is a versatile and highly regulated mechanism that enables cells to respond to a wide range of extracellular signals. Through the activation of G-proteins and the generation of second messengers, GPCRs orchestrate diverse cellular responses essential for physiological functions. Dysregulation of GPCR pathways is implicated in numerous diseases, making them critical targets for therapeutic interventions.
Q6. How do receptor tyrosine kinases (RTKs) initiate and propagate signals within the cell, and what are the key pathways activated by RTKs?
Answer:
Receptor Tyrosine Kinases (RTKs) Overview: RTKs are a class of transmembrane receptors that respond to extracellular signals, particularly growth factors, by initiating intracellular signaling cascades through their intrinsic tyrosine kinase activity. They play pivotal roles in regulating cell growth, differentiation, metabolism, and survival.
Mechanism of RTK Signal Initiation and Propagation:
Ligand Binding and Receptor Dimerization:
- Ligand Binding: An extracellular ligand (e.g., epidermal growth factor, insulin) binds to the extracellular domain of the RTK.
- Dimerization: Ligand binding induces the dimerization (pairing) of two RTK molecules, bringing their intracellular kinase domains into proximity.
Autophosphorylation:
- Kinase Activation: The juxtaposition of kinase domains facilitates trans-phosphorylation, where each RTK phosphorylates tyrosine residues on the other receptor.
- Phosphotyrosines: The phosphorylated tyrosines serve as docking sites for downstream signaling proteins containing SH2 (Src Homology 2) or PTB (Phosphotyrosine Binding) domains.
Recruitment of Adaptor Proteins and Enzymes:
- Adaptor Proteins: Proteins like Grb2 and Shc bind to phosphotyrosines, linking RTKs to other signaling molecules.
- Enzymes: Enzymes such as PI3K (Phosphoinositide 3-Kinase) and Src family kinases are recruited to the activated receptor complex.
Activation of Downstream Signaling Pathways:
- MAPK/ERK Pathway: Adaptor proteins link RTKs to the Ras-MAPK cascade, leading to the activation of ERK (Extracellular signal-Regulated Kinase), which translocates to the nucleus to regulate gene expression.
- PI3K/Akt Pathway: PI3K phosphorylates PIP₂ to generate PIP₃, which recruits and activates Akt (Protein Kinase B), promoting cell survival and growth.
- PLCγ Pathway: Phospholipase C gamma (PLCγ) is activated, leading to the production of IP₃ and DAG, which mobilize calcium ions and activate PKC, respectively.
- JAK/STAT Pathway: Some RTKs activate Janus kinases (JAKs), which phosphorylate STAT (Signal Transducer and Activator of Transcription) proteins. Phosphorylated STATs dimerize and translocate to the nucleus to modulate gene expression.
Signal Amplification and Integration:
- Cascade Effect: Each activated kinase can phosphorylate multiple downstream targets, amplifying the signal.
- Cross-Talk: RTK pathways can interact with other signaling pathways, integrating multiple signals to produce a coordinated cellular response.
Termination of Signal:
- Dephosphorylation: Protein tyrosine phosphatases remove phosphate groups from RTKs and downstream signaling proteins, inactivating the pathway.
- Endocytosis: Activated RTKs can be internalized through endocytosis, sequestering them away from signaling molecules and targeting them for degradation or recycling.
Key Pathways Activated by RTKs:
MAPK/ERK Pathway:
- Components: Ras → Raf → MEK → ERK
- Function: Regulates gene expression, cell division, differentiation, and survival.
- Example: EGF (Epidermal Growth Factor) binding to EGFR activates the MAPK pathway, promoting cell proliferation.
PI3K/Akt Pathway:
- Components: PI3K → PIP₃ → Akt → mTOR
- Function: Promotes cell growth, survival, metabolism, and angiogenesis.
- Example: Insulin binding to the insulin receptor activates PI3K/Akt signaling, enhancing glucose uptake and metabolism.
PLCγ Pathway:
- Components: PLCγ → IP₃ + DAG → Ca²⁺ release + PKC activation
- Function: Regulates intracellular calcium levels, cell proliferation, and differentiation.
- Example: VEGF (Vascular Endothelial Growth Factor) binding to its RTK activates PLCγ, promoting angiogenesis.
JAK/STAT Pathway:
- Components: JAKs → STATs → Gene Expression
- Function: Mediates responses to cytokines and growth factors, influencing immune function and cell growth.
- Example: Growth hormone binding to its RTK activates the JAK/STAT pathway, regulating growth and metabolism.
Consequences of RTK Dysregulation:
Cancer:
- Overactivation: Mutations leading to constitutive RTK activation can drive uncontrolled cell proliferation and survival.
- Examples: HER2 amplification in breast cancer, EGFR mutations in lung cancer.
Developmental Disorders:
- Impaired Signaling: Defects in RTK pathways can result in abnormal development, such as craniosynostosis due to FGFR mutations.
Metabolic Diseases:
- Insulin Resistance: Impaired RTK signaling through the insulin receptor contributes to type 2 diabetes.
Conclusion: Receptor tyrosine kinases are critical mediators of cellular responses to growth factors and other extracellular signals. By initiating and propagating multiple signaling cascades, RTKs regulate essential cellular processes such as growth, differentiation, and survival. Dysregulation of RTK signaling is implicated in various diseases, particularly cancer, making RTKs important targets for therapeutic interventions.
Q7. How do cells utilize calcium ions (Ca²⁺) as second messengers, and what are the diverse cellular processes regulated by Ca²⁺ signaling?
Answer:
Calcium Ions (Ca²⁺) as Second Messengers: Calcium ions (Ca²⁺) are versatile second messengers involved in a wide range of cellular signaling pathways. Their concentration within the cytoplasm is tightly regulated, allowing them to serve as dynamic signals that trigger various cellular responses upon transient increases in their levels.
Mechanisms of Ca²⁺ Signaling:
Calcium Release:
- IP₃ Receptors: Inositol 1,4,5-triphosphate (IP₃) binds to IP₃ receptors on the endoplasmic reticulum (ER), causing the release of Ca²⁺ into the cytoplasm.
- Ryanodine Receptors: Activated by calcium-induced calcium release (CICR), particularly in muscle cells, leading to a rapid and significant increase in cytoplasmic Ca²⁺.
- Store-Operated Calcium Entry (SOCE): Depletion of ER Ca²⁺ stores triggers the opening of plasma membrane channels (e.g., ORAI1) to allow extracellular Ca²⁺ influx.
Ca²⁺ Pumps and Exchangers:
- SERCA Pumps: Sarco/Endoplasmic Reticulum Ca²⁺-ATPase pumps Ca²⁺ back into the ER, restoring low cytoplasmic Ca²⁺ levels.
- PMCA Pumps: Plasma Membrane Ca²⁺-ATPase pumps Ca²⁺ out of the cell, maintaining low intracellular Ca²⁺ concentration.
- Na⁺/Ca²⁺ Exchangers: Use the sodium gradient to extrude Ca²⁺ from the cell.
Ca²⁺ Buffers and Binding Proteins:
- Calmodulin: Binds Ca²⁺ and activates various enzymes and proteins, including kinases and phosphatases.
- Calbindin and Parvalbumin: Bind Ca²⁺ to modulate its availability and prevent excessive cytoplasmic Ca²⁺ levels.
Diverse Cellular Processes Regulated by Ca²⁺ Signaling:
Muscle Contraction:
- Skeletal Muscle: Ca²⁺ binds to troponin, causing tropomyosin to shift and expose myosin-binding sites on actin filaments, enabling contraction.
- Cardiac Muscle: Ca²⁺-induced calcium release from the sarcoplasmic reticulum leads to coordinated heart muscle contractions.
- Smooth Muscle: Ca²⁺ binds to calmodulin, activating myosin light-chain kinase (MLCK) to phosphorylate myosin and initiate contraction.
Neurotransmitter Release:
- Synaptic Vesicle Fusion: In neurons, Ca²⁺ influx through voltage-gated calcium channels triggers the fusion of synaptic vesicles with the plasma membrane, releasing neurotransmitters into the synaptic cleft.
Gene Expression:
- Transcription Factor Activation: Ca²⁺-calmodulin complexes can activate transcription factors like CREB (cAMP response element-binding protein), influencing gene expression related to cell growth and differentiation.
Cell Motility and Cytoskeletal Dynamics:
- Actin Polymerization: Ca²⁺ regulates proteins involved in actin filament assembly and disassembly, affecting cell movement and shape changes.
- Rho GTPases Activation: Ca²⁺ can influence the activity of Rho family GTPases, which regulate the cytoskeleton and cell adhesion.
Apoptosis (Programmed Cell Death):
- Mitochondrial Pathway: Elevated Ca²⁺ levels can trigger the release of pro-apoptotic factors from mitochondria, initiating apoptosis.
- Calcium-Dependent Enzymes: Activation of calcium-dependent proteases like calpains can lead to apoptotic signaling.
Metabolic Regulation:
- Enzyme Activation: Ca²⁺ activates various metabolic enzymes, including those involved in glycolysis and the citric acid cycle, modulating cellular metabolism based on energy needs.
Immune Responses:
- T-Cell Activation: Ca²⁺ signaling is crucial for the activation of T-cells, leading to the production of cytokines and the proliferation of immune cells.
- Phagocytosis: Ca²⁺ regulates the engulfment and digestion of pathogens by phagocytic immune cells.
Sensory Transduction:
- Vision: In photoreceptor cells, Ca²⁺ levels regulate the phototransduction cascade, affecting visual signal processing.
- Hearing: Ca²⁺ modulates the function of ion channels in hair cells of the inner ear, influencing auditory signal transduction.
Regulation of Ca²⁺ Signaling:
- Spatial and Temporal Control: Localized Ca²⁺ release and rapid buffering ensure precise control over Ca²⁺ signals.
- Feedback Mechanisms: Ca²⁺ can activate mechanisms that reduce its own levels, preventing excessive signaling and maintaining homeostasis.
Consequences of Ca²⁺ Dysregulation:
- Cellular Damage: Excessive Ca²⁺ can activate destructive enzymes, leading to cell injury or death.
- Neurological Disorders: Impaired Ca²⁺ signaling is implicated in diseases like Alzheimer’s, Parkinson’s, and Huntington’s.
- Cardiac Arrhythmias: Abnormal Ca²⁺ handling in heart cells can disrupt normal heart rhythms.
Conclusion: Calcium ions are pivotal second messengers that regulate a multitude of cellular processes through tightly controlled signaling pathways. Their ability to rapidly and reversibly modulate various targets makes Ca²⁺ essential for cellular communication, adaptation, and function. Understanding Ca²⁺ signaling mechanisms is crucial for elucidating cellular behavior and developing interventions for diseases associated with Ca²⁺ dysregulation.
Q7. How do cells maintain specificity in their responses to multiple simultaneous signals, and what role do scaffolding proteins play in achieving this specificity?
Answer:
Maintaining Specificity in Cellular Responses: Cells are exposed to a multitude of signaling molecules simultaneously, each initiating different signaling pathways. Maintaining specificity ensures that the correct cellular response is elicited without cross-activation of unrelated pathways. This specificity is achieved through several mechanisms:
Receptor Specificity:
- Unique Ligand-Receptor Interactions: Each receptor is specific to its ligand, ensuring that only the appropriate signaling pathways are activated.
- Receptor Localization: Receptors are strategically located within specific membrane domains (e.g., lipid rafts) to facilitate selective signaling.
Spatial and Temporal Regulation:
- Compartmentalization: Signaling components are localized to specific areas within the cell, preventing unintended interactions.
- Temporal Control: Signals are activated and terminated in a timely manner to prevent overlap and interference between pathways.
Signal Amplification and Feedback:
- Controlled Amplification: Signal amplification occurs within defined pathways, limiting the spread to unrelated pathways.
- Feedback Inhibition: Negative feedback loops ensure that signals are terminated appropriately, maintaining pathway integrity.
Molecular Docking Sites:
- Specific Protein-Protein Interactions: Proteins within a signaling pathway have specific domains (e.g., SH2, SH3) that mediate precise interactions, ensuring pathway fidelity.
Role of Scaffolding Proteins in Achieving Specificity:
Definition and Function: Scaffolding proteins are multi-domain proteins that serve as platforms to organize and assemble specific signaling complexes. They bring together multiple components of a signaling pathway in close proximity, facilitating efficient and specific signal transduction.
Mechanisms by Which Scaffolding Proteins Enhance Specificity:
Spatial Organization:
- Localizing Signaling Components: Scaffolding proteins position kinases, phosphatases, and other signaling molecules at specific locations within the cell, ensuring that interactions occur only among designated partners.
- Preventing Crosstalk: By segregating different signaling pathways, scaffolding proteins minimize unintended interactions between components of unrelated pathways.
Temporal Coordination:
- Sequential Activation: Scaffolding proteins facilitate the orderly progression of signaling events, ensuring that each step is completed before the next begins.
- Regulating Signal Duration: They can also influence the duration of signaling by controlling the accessibility and activity of pathway components.
Efficiency and Signal Amplification:
- Enhanced Interaction Rates: Proximity of signaling molecules increases the likelihood of their interactions, making signal transduction more efficient.
- Multivalent Binding: Scaffolding proteins often contain multiple binding sites, allowing them to simultaneously bind several signaling proteins, thereby amplifying the signal within the pathway.
Integration of Multiple Signals:
- Converging Pathways: Some scaffolding proteins can integrate signals from multiple receptors, coordinating complex cellular responses.
- Feedback Regulation: They can incorporate feedback mechanisms that fine-tune the signaling output based on cellular conditions.
Examples of Scaffolding Proteins:
KSR (Kinase Suppressor of Ras):
- Function: Organizes components of the MAPK/ERK pathway, including Raf, MEK, and ERK, facilitating efficient signal transduction from Ras to ERK.
Axin:
- Function: Serves as a scaffolding protein in the Wnt signaling pathway, bringing together components like β-catenin, GSK-3β, and APC to regulate β-catenin stability.
Ste5:
- Function: In yeast, Ste5 scaffolds components of the mating pheromone response pathway, ensuring specific activation of downstream kinases.
JIP (JNK-Interacting Protein):
- Function: Scaffolds components of the JNK signaling pathway, promoting specific activation of JNK in response to stress signals.
Consequences of Scaffolding Protein Dysfunction:
Loss of Specificity:
- Crosstalk and Misregulation: Without proper scaffolding, signaling components may interact with unintended partners, leading to aberrant signaling and cellular responses.
Impaired Signal Transduction:
- Reduced Efficiency: Disruption of scaffolding can decrease the efficiency of signal transmission, weakening the cellular response to stimuli.
Disease Association:
- Cancer and Developmental Disorders: Mutations or dysregulation of scaffolding proteins can contribute to uncontrolled cell growth, impaired differentiation, and other pathological conditions.
Conclusion: Scaffolding proteins are essential for maintaining specificity in cellular responses by organizing and coordinating signaling complexes. They ensure that signaling pathways operate with precision, preventing unwanted interactions and enhancing the efficiency of signal transduction. Understanding the roles of scaffolding proteins provides insights into the complexity of cellular communication and the mechanisms underlying various diseases.
Q8. What is the significance of feedback loops in cell signaling pathways, and how do negative and positive feedback mechanisms influence cellular responses?
Answer:
Significance of Feedback Loops in Cell Signaling Pathways: Feedback loops are regulatory mechanisms where the output of a process influences its own activity, either enhancing or inhibiting it. In cell signaling pathways, feedback loops are crucial for maintaining homeostasis, controlling the intensity and duration of signals, and ensuring appropriate cellular responses to stimuli.
Types of Feedback Loops:
Negative Feedback:
- Definition: The output of a process inhibits its own production, acting as a brake to prevent overactivation.
- Function: Maintains homeostasis by stabilizing cellular conditions and ensuring signals do not become excessively strong or prolonged.
- Examples:
- cAMP Pathway: High levels of cAMP activate phosphodiesterase, which degrades cAMP, reducing PKA activity and dampening the signal.
- Hormonal Regulation: Elevated thyroid hormone levels inhibit the release of thyrotropin-releasing hormone (TRH) and thyroid-stimulating hormone (TSH), preventing overproduction of thyroid hormones.
Positive Feedback:
- Definition: The output of a process enhances its own production, acting as an amplifier to drive processes to completion.
- Function: Facilitates rapid and decisive cellular responses, ensuring that certain events are fully carried out.
- Examples:
- Blood Clotting: Activation of clotting factors leads to the conversion of more clotting factors, rapidly forming a blood clot.
- Ovulation: Increased levels of luteinizing hormone (LH) trigger the release of more LH, leading to the rupture of the ovarian follicle.
Influence of Feedback Mechanisms on Cellular Responses:
Negative Feedback:
- Signal Termination: Prevents prolonged signaling by inhibiting the pathway once the desired response is achieved.
- Stabilization: Maintains stable internal environments by counteracting deviations from equilibrium.
- Example: In the insulin signaling pathway, increased glucose uptake and metabolism activate mechanisms that reduce insulin secretion, preventing hypoglycemia.
Effects:
- Homeostasis: Ensures balanced cellular activities.
- Prevention of Overstimulation: Avoids excessive cellular responses that could be detrimental.
- Temporal Control: Regulates the duration of signaling, allowing transient responses when necessary.
Positive Feedback:
- Signal Amplification: Enhances the strength and speed of the signal, driving processes to completion.
- Irreversible Decisions: Facilitates decisive cellular actions, such as cell division or differentiation, by committing to a particular pathway.
- Example: During cytokinesis, the formation of the contractile ring is reinforced by positive feedback mechanisms that promote further contraction until the cell splits.
Effects:
- Rapid Responses: Enables swift and robust cellular actions.
- Threshold Crossing: Helps cells to quickly reach the necessary levels of signaling for a full response.
- Potential for Runaway Signals: If not properly regulated, positive feedback can lead to uncontrolled signaling and pathological conditions.
Regulation of Feedback Loops:
Integration with Other Pathways:
- Feedback loops often interact with other signaling pathways to provide coordinated regulation and prevent conflicts between signals.
Temporal Dynamics:
- The timing of feedback activation is critical. Negative feedback is typically activated after the initial signal to prevent overshooting, while positive feedback is activated early to amplify the response.
Scaffold Proteins and Compartmentalization:
- Localization of signaling components can influence the effectiveness and specificity of feedback loops, ensuring that feedback affects only targeted pathways.
Consequences of Feedback Loop Dysregulation:
Excessive Negative Feedback:
- Insufficient Responses: May dampen necessary signals, leading to inadequate cellular responses to stimuli.
- Example: Overactivation of negative feedback in immune signaling can suppress immune responses, increasing susceptibility to infections.
Excessive Positive Feedback:
- Runaway Signaling: Can result in excessive cellular responses, contributing to diseases such as cancer, where positive feedback loops promote uncontrolled cell proliferation.
- Example: Constitutive activation of positive feedback in the Ras-MAPK pathway can drive continuous cell division and tumorigenesis.
Conclusion: Feedback loops are essential for the precise regulation of cell signaling pathways, ensuring that cellular responses are appropriate, timely, and controlled. Negative feedback maintains homeostasis and prevents overstimulation, while positive feedback drives robust and irreversible cellular processes. Proper functioning of these feedback mechanisms is crucial for cellular health, and their dysregulation is often associated with various diseases, highlighting the importance of understanding feedback dynamics in cell biology.
Q9. Describe the mechanisms by which cells communicate through direct contact, and provide examples of processes that utilize contact-dependent signaling.
Answer:
Direct Contact Communication Overview: Cells can communicate through direct physical interactions, requiring cell-to-cell contact. This mode of communication is essential for maintaining tissue integrity, coordinating cellular activities, and regulating developmental processes. Contact-dependent signaling involves membrane-bound ligands and receptors, allowing precise and localized signal transmission.
Mechanisms of Contact-Dependent Signaling:
Juxtacrine Signaling:
- Definition: A form of cell signaling where the signal-emitting and signal-receiving cells are in direct contact.
- Components: Membrane-bound ligands on one cell interact with membrane-bound receptors on an adjacent cell.
- Types:
- Signaling through Direct Membrane Proteins: Ligands and receptors remain attached to the membranes of their respective cells during signaling.
- Gap Junction Communication: Direct cytoplasmic connections between cells allow the passage of ions and small molecules.
Contact-Mediated Induction:
- Definition: A process where cell fate is determined through direct contact with neighboring cells.
- Function: Critical in embryonic development and tissue differentiation, ensuring that cells develop specific identities based on their position and interactions.
Neural Synapses:
- Definition: Specialized junctions where neurons communicate with other neurons, muscle cells, or glands.
- Mechanism: Electrical or chemical synapses facilitate rapid and specific signal transmission across the synaptic cleft.
Immunological Synapses:
- Definition: Structured interfaces between T cells and antigen-presenting cells (APCs).
- Function: Enable precise recognition of antigens and activation of immune responses through the clustering of receptors and signaling molecules.
Examples of Processes Utilizing Contact-Dependent Signaling:
Developmental Patterning:
- Notch Signaling Pathway:
- Mechanism: Interaction between Notch receptors on one cell and Delta/Jagged ligands on an adjacent cell.
- Function: Influences cell differentiation, proliferation, and apoptosis during embryonic development.
- Example: Determination of neuronal and glial cell fate in the nervous system.
- Notch Signaling Pathway:
Immune Cell Activation:
- T-Cell Activation:
- Mechanism: T cell receptors (TCRs) on T cells recognize antigens presented by MHC molecules on APCs.
- Function: Triggers T cell activation, proliferation, and differentiation into effector cells.
- Example: Activation of helper T cells by dendritic cells presenting foreign antigens.
- T-Cell Activation:
Cell Adhesion and Tissue Formation:
- Cadherin-Mediated Adhesion:
- Mechanism: Cadherin proteins on adjacent cells interact through homophilic binding, linking cells together.
- Function: Maintains tissue structure and integrity by mediating cell-cell adhesion.
- Example: Formation and maintenance of epithelial layers through E-cadherin interactions.
- Cadherin-Mediated Adhesion:
Muscle Contraction:
- Neuromuscular Junctions:
- Mechanism: Motor neurons form synapses with muscle fibers, releasing neurotransmitters like acetylcholine.
- Function: Induces muscle fiber depolarization and contraction.
- Example: Rapid communication between motor neurons and skeletal muscle fibers to facilitate movement.
- Neuromuscular Junctions:
Stem Cell Niche Maintenance:
- Hedgehog Signaling in Stem Cell Niches:
- Mechanism: Hedgehog ligands are presented on niche cells and interact with receptors on stem cells.
- Function: Regulates stem cell maintenance, self-renewal, and differentiation.
- Example: Maintenance of hematopoietic stem cells in the bone marrow niche.
- Hedgehog Signaling in Stem Cell Niches:
Apoptosis Induction:
- Fas-Fas Ligand Interaction:
- Mechanism: Fas ligand (FasL) on one cell binds to Fas receptor on another cell.
- Function: Triggers apoptosis in the Fas-expressing cell, important for immune regulation and eliminating damaged cells.
- Example: Induction of apoptosis in infected or cancerous cells by cytotoxic T lymphocytes.
- Fas-Fas Ligand Interaction:
Synaptic Plasticity:
- Neuronal Synapse Formation and Remodeling:
- Mechanism: Neurons form synapses through direct contact, allowing the establishment and modification of neural networks.
- Function: Essential for learning, memory, and adaptation of the nervous system.
- Example: Formation of new synaptic connections in response to learning stimuli.
- Neuronal Synapse Formation and Remodeling:
Conclusion: Contact-dependent signaling is a fundamental mode of cellular communication that ensures precise and coordinated responses in multicellular organisms. Through direct interactions between membrane-bound ligands and receptors, cells can regulate a wide array of processes, including development, immune responses, tissue integrity, and specialized functions like muscle contraction and neuronal communication. Understanding these mechanisms is crucial for comprehending how complex tissues and organs are organized and maintained.
Q10. How do cells integrate multiple signaling pathways to produce a coordinated response, and what factors influence the outcome of such integration?
Answer:
Integration of Multiple Signaling Pathways Overview: Cells often receive and process multiple signals simultaneously, requiring the integration of various signaling pathways to produce a coherent and appropriate response. This integration ensures that cellular responses are finely tuned to the complex and dynamic environments in which cells operate.
Mechanisms of Signal Integration:
Convergence Points:
- Shared Components: Different signaling pathways may converge on common downstream effectors, allowing multiple inputs to regulate the same cellular processes.
- Example: Both the MAPK/ERK pathway and the PI3K/Akt pathway can influence gene expression through the activation of transcription factors like CREB.
Divergence Points:
- Branching Pathways: A single signaling molecule can activate multiple downstream pathways, leading to diverse cellular responses.
- Example: Activation of RTKs can simultaneously trigger the MAPK/ERK pathway for proliferation and the PI3K/Akt pathway for survival.
Cross-Talk Between Pathways:
- Direct Interactions: Components of one pathway can directly interact with components of another, modulating the activity of both pathways.
- Indirect Interactions: Pathways can influence each other through intermediate molecules or feedback loops.
- Example: The Wnt signaling pathway can interact with the PI3K/Akt pathway to regulate cell proliferation and survival.
Modular Design:
- Pathway Modules: Signaling pathways consist of distinct modules that can be independently regulated, allowing for flexible integration based on cellular context.
- Example: Scaffold proteins can organize specific subsets of signaling components, enabling selective pathway activation.
Temporal Dynamics:
- Sequential Activation: Pathways can be activated in a specific temporal order, ensuring that earlier signals influence the interpretation and response to later signals.
- Example: Initial activation of a kinase cascade can prime cells to respond to subsequent signals by modifying transcription factors.
Spatial Localization:
- Compartmentalization: Signaling components localized to specific cellular regions (e.g., lipid rafts, endosomes) can selectively interact with certain pathways.
- Example: Signals initiated at the plasma membrane may differ from those initiated from endosomal compartments, leading to distinct outcomes.
Factors Influencing the Outcome of Signal Integration:
Signal Strength and Duration:
- Threshold Effects: The intensity of each signal can determine whether a particular pathway is activated or remains dormant.
- Duration: Prolonged versus transient signals can lead to different cellular outcomes, such as differentiation versus proliferation.
Receptor Density and Distribution:
- Availability of Receptors: The number and distribution of receptors on the cell surface can influence the responsiveness to different signals.
- Example: High density of growth factor receptors may enhance sensitivity to mitogenic signals.
Scaffold and Adaptor Proteins:
- Organization of Signaling Complexes: Scaffold proteins facilitate the assembly of specific signaling complexes, ensuring that only relevant pathways are activated.
- Example: The scaffolding protein KSR organizes components of the MAPK pathway, promoting efficient signal transmission.
Feedback Mechanisms:
- Regulation of Pathway Activity: Positive and negative feedback loops can modulate the activity and integration of signaling pathways.
- Example: Negative feedback in the MAPK pathway can limit signal duration, allowing other pathways to influence the final response.
Post-Translational Modifications:
- Protein Modifications: Phosphorylation, ubiquitination, and other modifications can alter the activity, localization, or interactions of signaling proteins, affecting pathway integration.
- Example: Phosphorylation of a transcription factor by one pathway can influence its ability to be activated by another pathway.
Cellular Context and State:
- Differentiation Status: Stem cells versus differentiated cells may respond differently to the same signals due to variations in signaling machinery.
- Metabolic State: Nutrient availability and energy status can influence how signals are interpreted and integrated.
Competition for Signaling Components:
- Resource Allocation: Multiple pathways may compete for the same signaling molecules, affecting the overall outcome based on pathway priority and availability.
- Example: Competing activation of Raf by different upstream signals can influence the strength and specificity of the MAPK response.
Examples of Signal Integration:
Cell Cycle Regulation:
- Growth Factor and DNA Damage Signals: Integration of mitogenic signals (e.g., via RTKs) promoting cell cycle progression and stress signals (e.g., via p53) inducing cell cycle arrest or apoptosis.
- Outcome: Decision to proceed with division, repair DNA, or undergo apoptosis based on the balance of signals.
Immune Cell Activation:
- Antigen Recognition and Co-Stimulation: T cells integrate signals from the T cell receptor (antigen recognition) and co-stimulatory receptors (e.g., CD28) to fully activate immune responses.
- Outcome: Activation of T cells leads to proliferation, cytokine production, and differentiation into effector cells.
Neuronal Plasticity:
- Synaptic Activity and Growth Factors: Neurons integrate electrical signals from synaptic activity with growth factor signals to regulate synaptic strength and dendritic spine formation.
- Outcome: Modulation of synaptic connections underlying learning and memory.
Conclusion: Cells employ intricate mechanisms to integrate multiple signaling pathways, ensuring that responses are precise, coordinated, and appropriate to the cellular context. Factors such as signal strength, temporal dynamics, spatial localization, and the presence of scaffolding proteins play pivotal roles in determining the outcome of signal integration. Understanding how cells integrate signals is fundamental to elucidating complex biological processes and addressing dysregulated signaling in diseases.
Q11. How does receptor desensitization occur in GPCR signaling, and what are the physiological implications of this process?
Answer:
Receptor Desensitization in GPCR Signaling: Receptor desensitization is a regulatory mechanism that reduces the responsiveness of G-Protein Coupled Receptors (GPCRs) to continuous or repeated stimulation by ligands. This process prevents overstimulation, maintains cellular homeostasis, and ensures that cells can respond appropriately to varying signal strengths.
Mechanisms of Receptor Desensitization:
Phosphorylation of GPCRs:
- Role of GRKs (GPCR Kinases): GRKs specifically phosphorylate activated GPCRs on serine and threonine residues within the receptor’s cytoplasmic domains.
- Effect of Phosphorylation: Phosphorylation decreases the receptor’s affinity for G-proteins, reducing its ability to activate downstream signaling pathways.
Binding of Arrestins:
- Function of Arrestins: Arrestins (e.g., β-arrestin) bind to phosphorylated GPCRs, blocking further G-protein coupling.
- Roles of Arrestins:
- Prevent G-Protein Activation: Inhibit the receptor’s ability to activate G-proteins, effectively silencing the signal.
- Receptor Internalization: Facilitate the internalization of GPCRs through clathrin-coated pits, removing them from the cell surface and sequestering them for either recycling or degradation.
- β-Arrestin-Mediated Signaling: Arrestins can initiate alternative signaling pathways independent of G-proteins, contributing to diverse cellular responses.
Receptor Internalization and Downregulation:
- Endocytosis: Phosphorylated and arrestin-bound receptors are internalized via endocytosis, removing them from the plasma membrane.
- Recycling vs. Degradation:
- Recycling: Receptors are returned to the cell surface for potential reuse.
- Degradation: Receptors are targeted for lysosomal degradation, leading to a decrease in receptor numbers and prolonged desensitization.
Receptor Downregulation:
- Chronic Desensitization: Prolonged exposure to high ligand concentrations can lead to reduced expression of GPCRs on the cell surface through transcriptional and translational mechanisms.
- Example: Chronic exposure to high levels of epinephrine can downregulate β-adrenergic receptors, decreasing cell sensitivity to the hormone.
Physiological Implications of Receptor Desensitization:
Prevention of Overstimulation:
- Homeostasis Maintenance: Desensitization prevents excessive cellular responses to continuous or high levels of signaling molecules, protecting cells from potential damage due to overstimulation.
- Example: Desensitization of β-adrenergic receptors in the heart prevents excessive heart rate and contractility in response to prolonged epinephrine exposure.
Facilitation of Receptor Resensitization:
- Recovery of Responsiveness: Internalized receptors can be dephosphorylated and recycled back to the plasma membrane, restoring their ability to respond to new signals once the ligand concentration decreases.
- Example: After a brief exposure to high levels of a neurotransmitter, receptors can return to the cell surface and regain sensitivity for subsequent signaling events.
Regulation of Signal Termination:
- Controlled Signal Duration: Desensitization ensures that signaling pathways are not perpetually active, allowing cells to terminate responses when signals are no longer needed.
- Example: Termination of visual signals in photoreceptor cells after exposure to bright light through rhodopsin desensitization.
Adaptation to Sustained Stimuli:
- Cellular Adaptation: Cells adapt to persistent stimuli by desensitizing receptors, enabling them to modulate their sensitivity based on the environment.
- Example: Receptor desensitization in airway smooth muscle cells in response to chronic exposure to high levels of β-agonists, affecting bronchial tone regulation.
Implications in Drug Tolerance:
- Pharmacological Tolerance: Repeated use of drugs targeting GPCRs can lead to desensitization, requiring higher doses to achieve the same therapeutic effect.
- Example: Tolerance to β-adrenergic agonists used in asthma treatment due to receptor desensitization.
Contribution to Disease States:
- Receptor Dysfunction: Dysregulation of desensitization processes can contribute to various diseases, including heart failure, where impaired receptor desensitization affects cardiac function.
- Neurological Disorders: Altered desensitization of neurotransmitter receptors can influence synaptic plasticity and contribute to conditions like addiction and depression.
Conclusion: Receptor desensitization is a critical regulatory mechanism in GPCR signaling that ensures cells respond appropriately to varying levels of stimuli. By modulating receptor activity and availability, desensitization maintains cellular homeostasis, prevents overstimulation, and allows cells to adapt to changing environments. Understanding desensitization mechanisms is essential for developing therapeutic strategies that manage drug tolerance and address diseases associated with receptor dysregulation.
Q12. How do scaffolding proteins enhance the specificity and efficiency of signal transduction pathways, and what are the consequences of their malfunction?
Answer:
Scaffolding Proteins Overview: Scaffolding proteins are multi-domain proteins that organize and assemble specific signaling complexes by bringing together multiple components of a signaling pathway. They play a crucial role in enhancing the specificity and efficiency of signal transduction by ensuring that signaling molecules interact in a controlled and organized manner.
Enhancement of Specificity and Efficiency:
Spatial Organization:
- Localization of Signaling Components: Scaffolding proteins localize kinases, phosphatases, and other signaling molecules to specific cellular compartments, ensuring that interactions occur only among designated partners.
- Example: KSR (Kinase Suppressor of Ras) scaffolds components of the MAPK/ERK pathway, ensuring that Raf, MEK, and ERK interact efficiently.
Signal Amplification:
- Proximity Effect: By physically bringing signaling molecules closer together, scaffolding proteins increase the likelihood of productive interactions, amplifying the signal.
- Example: Scaffold proteins in the JNK signaling pathway facilitate rapid phosphorylation and activation of JNK.
Prevention of Crosstalk:
- Pathway Segregation: Scaffolding proteins segregate different signaling pathways, minimizing unintended interactions and maintaining pathway fidelity.
- Example: Axin scaffolds components of the Wnt signaling pathway, preventing interference with other signaling cascades.
Temporal Coordination:
- Sequential Activation: Scaffolding proteins can coordinate the timing of signaling events, ensuring that each step of the pathway is activated in the correct order.
- Example: Ste5 in yeast organizes the sequential activation of kinases in the pheromone response pathway, ensuring timely and coordinated cellular responses.
Integration of Multiple Signals:
- Converging Inputs: Some scaffolding proteins can integrate signals from multiple receptors or pathways, enabling complex and nuanced cellular responses.
- Example: Certain scaffold proteins can bind to different signaling modules, allowing integration of growth factor and stress signals to modulate cell fate decisions.
Facilitation of Feedback Regulation:
- Incorporation of Feedback Loops: Scaffolding proteins can incorporate feedback inhibitors or activators within the signaling complex, enabling dynamic regulation of the pathway.
- Example: Scaffold proteins can recruit phosphatases that dephosphorylate and inactivate kinases, providing negative feedback control.
Consequences of Scaffolding Protein Malfunction:
Loss of Signal Specificity:
- Increased Crosstalk: Without proper scaffolding, signaling molecules from different pathways may interact inappropriately, leading to mixed or unintended signals.
- Example: Disruption of KSR-mediated MAPK pathway assembly can result in aberrant activation of ERK by unrelated kinases.
Reduced Signal Efficiency:
- Inefficient Signaling: Absence or malfunction of scaffolding proteins can lead to decreased interaction rates among signaling components, weakening the overall signal.
- Example: Loss of Ste5 scaffolding in yeast impairs the pheromone response, reducing the cell’s ability to respond to mating signals.
Altered Signal Dynamics:
- Timing Disruptions: Malfunctioning scaffolding proteins can disrupt the temporal coordination of signaling events, leading to delayed or inappropriate cellular responses.
- Example: Impaired scaffolding in the Wnt pathway can affect the timing of β-catenin stabilization, altering gene expression patterns.
Disease Association:
- Cancer: Overactive scaffolding proteins can lead to persistent signaling pathways that drive uncontrolled cell proliferation and survival.
- Neurodegenerative Diseases: Impaired scaffolding in neuronal signaling pathways can disrupt synaptic function and neuronal health.
- Example: Mutations in axin scaffolding proteins are associated with colorectal cancer due to dysregulated Wnt signaling.
Developmental Defects:
- Impaired Tissue Formation: Scaffolding protein malfunctions can disrupt developmental signaling pathways, leading to congenital abnormalities and impaired tissue differentiation.
- Example: Defects in scaffold proteins involved in Hedgehog signaling can result in developmental disorders like holoprosencephaly.
Impaired Immune Responses:
- Dysregulated Signaling: Scaffolding protein malfunctions can affect immune cell activation and responses, compromising the immune system’s ability to fight infections.
- Example: Defective scaffolding in T-cell receptor signaling can impair T-cell activation and immune function.
Conclusion: Scaffolding proteins are essential for the precise and efficient operation of signal transduction pathways. By organizing and coordinating signaling complexes, they ensure that cellular responses are specific, robust, and appropriately regulated. Malfunctions in scaffolding proteins can lead to a wide range of biological dysfunctions and diseases, underscoring their critical role in maintaining cellular and organismal health. Understanding scaffolding protein mechanisms provides valuable insights into cellular signaling intricacies and offers potential targets for therapeutic interventions in various diseases.
Conclusion: These thought-provoking questions and detailed answers encompass fundamental aspects of cell communication, including signaling types, receptor functions, signal transduction mechanisms, second messengers, pathway integration, feedback regulation, contact-dependent signaling, and the role of scaffolding proteins. They are designed to deepen understanding, encourage critical thinking, and reinforce key concepts essential for mastering cell biology. Utilizing these questions as study aids can help learners grasp the complex mechanisms of cellular communication and appreciate the intricacies of signal regulation within living organisms.
Cell Communication and Signaling: Thought-Provoking Questions
1. What are the primary types of cell signaling, and how does each type facilitate communication between cells in different spatial contexts?
Answer:
Cell signaling is essential for coordinating activities within multicellular organisms. The primary types of cell signaling are autocrine, paracrine, endocrine, and juxtacrine signaling. Each type operates over different spatial ranges and facilitates communication in distinct contexts:
Autocrine Signaling:
- Definition: Cells respond to signaling molecules that they themselves produce.
- Function: Enables cells to regulate their own behavior, such as growth, differentiation, or apoptosis.
- Spatial Context: Acts on the same cell that secretes the signaling molecule.
- Example: T cells in the immune system release cytokines that bind to receptors on their own surface to promote their activation and proliferation.
Paracrine Signaling:
- Definition: Cells communicate by releasing signaling molecules that affect nearby target cells.
- Function: Facilitates localized responses, such as coordinating tissue growth or immune responses.
- Spatial Context: Acts on adjacent or nearby cells within the same tissue.
- Example: Neurotransmitters released by neurons diffuse across synapses to bind receptors on adjacent neurons or muscle cells, enabling nerve signal transmission.
Endocrine Signaling:
- Definition: Cells release hormones into the bloodstream, which travel long distances to reach target cells throughout the body.
- Function: Regulates physiological processes on a systemic level, such as metabolism, growth, and reproduction.
- Spatial Context: Acts on distant target cells across the entire organism.
- Example: The pancreas secretes insulin into the blood, which travels to various tissues (e.g., liver, muscle) to regulate glucose uptake and metabolism.
Juxtacrine (Contact-Dependent) Signaling:
- Definition: Direct communication between adjacent cells through cell-to-cell contact.
- Function: Critical for processes requiring precise spatial organization, such as embryonic development and immune cell interactions.
- Spatial Context: Requires direct physical contact between signaling and target cells.
- Example: Notch signaling involves the interaction of Notch receptors on one cell with Delta ligands on an adjacent cell, influencing cell fate decisions during development.
Summary:
- Autocrine: Self-regulation, same cell.
- Paracrine: Local communication, nearby cells.
- Endocrine: Systemic regulation, distant cells via bloodstream.
- Juxtacrine: Direct contact, adjacent cells.
Understanding these signaling types is fundamental for comprehending how cells coordinate complex physiological functions, maintain tissue integrity, and respond to environmental changes.
2. How do G-Protein Coupled Receptors (GPCRs) and Receptor Tyrosine Kinases (RTKs) differ in their structure and mechanisms of signal transduction?
Answer:
G-Protein Coupled Receptors (GPCRs) and Receptor Tyrosine Kinases (RTKs) are two major classes of cell surface receptors involved in signal transduction. They differ significantly in their structure, ligand specificity, and intracellular signaling mechanisms.
1. Structure:
GPCRs:
- Transmembrane Domains: Seven transmembrane α-helices.
- Ligand Binding: Bind a diverse range of ligands, including hormones, neurotransmitters, and sensory stimuli.
- Intracellular Components: Associated with heterotrimeric G-proteins (composed of α, β, and γ subunits).
RTKs:
- Transmembrane Domains: Single-pass transmembrane proteins.
- Ligand Binding: Typically bind large protein ligands, such as growth factors.
- Intracellular Components: Possess intrinsic tyrosine kinase domains that phosphorylate specific tyrosine residues upon activation.
2. Mechanism of Signal Transduction:
GPCRs:
- Ligand Binding: A ligand binds to the extracellular domain of the GPCR.
- Conformational Change: Induces a conformational change in the receptor.
- G-Protein Activation: The activated GPCR interacts with a G-protein, causing the exchange of GDP for GTP on the Gα subunit.
- Signal Propagation: The Gα-GTP and Gβγ subunits dissociate and interact with downstream effectors (e.g., adenylyl cyclase, phospholipase C).
- Second Messenger Production: Activation of effectors leads to the production of second messengers like cAMP, IP₃, and DAG, which further propagate the signal.
RTKs:
- Ligand Binding: A ligand binds to the extracellular domain of the RTK, typically inducing dimerization (pairing of two receptor molecules).
- Autophosphorylation: The intracellular tyrosine kinase domains phosphorylate each other on specific tyrosine residues.
- Docking Site Creation: Phosphorylated tyrosines serve as docking sites for adaptor proteins and other signaling molecules containing SH2 or PTB domains.
- Signal Propagation: Recruitment of these proteins initiates various signaling cascades, such as the MAPK/ERK pathway or the PI3K/Akt pathway.
- Cellular Response: These cascades lead to changes in gene expression, cell growth, differentiation, or survival.
3. Ligand Specificity and Diversity:
- GPCRs: Highly diverse in both structure and ligand specificity, allowing them to respond to a wide array of signals.
- RTKs: More uniform in structure, primarily responding to growth factors and related proteins.
4. Signal Amplification:
- GPCRs: Amplification occurs through the G-protein cycle; one activated GPCR can activate multiple G-proteins, each generating multiple second messengers.
- RTKs: Amplification is achieved through kinase cascades; each phosphorylated RTK can recruit multiple adaptor proteins, each initiating multiple downstream signaling events.
5. Functional Roles:
- GPCRs: Involved in sensory perception (e.g., vision, taste, smell), neurotransmission, immune responses, and hormonal regulation.
- RTKs: Crucial for regulating cell growth, differentiation, metabolism, and survival; often implicated in developmental processes and cancer.
Conclusion: GPCRs and RTKs are pivotal for cellular communication but operate through distinct structural and mechanistic pathways. GPCRs offer versatility in ligand recognition and rapid signal transduction through G-proteins and second messengers, while RTKs provide robust and sustained signaling through kinase cascades essential for growth and differentiation. Understanding their differences is fundamental for targeting these receptors in various therapeutic interventions.
3. What roles do second messengers play in signal transduction pathways, and how do specific second messengers such as cAMP, IP₃, DAG, and Ca²⁺ contribute to diverse cellular responses?
Answer:
Second Messengers in Signal Transduction: Second messengers are intracellular signaling molecules that relay and amplify signals received by cell surface receptors. They play crucial roles in translating extracellular signals into specific intracellular responses, ensuring that cells can rapidly and effectively respond to various stimuli.
Key Roles of Second Messengers:
- Signal Amplification: A single activated receptor can generate multiple second messenger molecules, amplifying the initial signal.
- Divergence: They can activate multiple downstream targets, allowing for diverse cellular responses.
- Integration: Second messengers can integrate signals from different pathways, coordinating complex cellular functions.
- Spatial and Temporal Regulation: They can diffuse rapidly within the cell, providing precise control over the location and duration of the signal.
Specific Second Messengers and Their Functions:
Cyclic Adenosine Monophosphate (cAMP):
- Generation: Produced by adenylyl cyclase from ATP upon activation by Gαs subunits of G-proteins or by certain RTKs.
- Function:
- Activation of Protein Kinase A (PKA): cAMP binds to the regulatory subunits of PKA, releasing and activating the catalytic subunits.
- Phosphorylation of Targets: PKA phosphorylates various proteins, regulating processes such as metabolism, gene expression, and ion channel activity.
- Example: In liver cells, cAMP-PKA pathway promotes glycogen breakdown in response to adrenaline.
Inositol 1,4,5-Triphosphate (IP₃) and Diacylglycerol (DAG):
- Generation: Produced by phospholipase C (PLC) cleavage of phosphatidylinositol 4,5-bisphosphate (PIP₂).
- Function:
- IP₃:
- Ca²⁺ Release: Binds to IP₃ receptors on the endoplasmic reticulum (ER), triggering the release of Ca²⁺ into the cytoplasm.
- Regulation of Ca²⁺-Dependent Processes: Influences muscle contraction, secretion, metabolism, and gene expression.
- DAG:
- Activation of Protein Kinase C (PKC): Remains in the membrane and activates PKC, which phosphorylates target proteins involved in cell growth, differentiation, and apoptosis.
- IP₃:
- Example: In pancreatic cells, IP₃ and DAG mediate insulin secretion in response to high blood glucose levels.
Calcium Ions (Ca²⁺):
- Generation: Released from intracellular stores (e.g., ER) via IP₃ receptors or voltage-gated calcium channels; also enters the cell through store-operated calcium entry (SOCE) channels.
- Function:
- Activation of Calcium-Binding Proteins: Such as calmodulin, which can activate various enzymes and kinases.
- Regulation of Cellular Processes: Including muscle contraction, neurotransmitter release, gene expression, and cell motility.
- Example: In neurons, Ca²⁺ influx triggers the release of neurotransmitters at synaptic terminals.
Cyclic Guanosine Monophosphate (cGMP):
- Generation: Produced by guanylyl cyclase from GTP, activated by nitric oxide (NO) or natriuretic peptides.
- Function:
- Activation of Protein Kinase G (PKG): cGMP binds to PKG, leading to phosphorylation of target proteins.
- Regulation of Smooth Muscle Relaxation: Promotes vasodilation and blood flow.
- Example: In vascular smooth muscle cells, cGMP-PKG pathway induces relaxation, lowering blood pressure.
Integration and Crosstalk: Second messengers often interact with multiple signaling pathways, allowing cells to integrate diverse signals and mount coordinated responses. For instance, Ca²⁺ can activate both calmodulin-dependent kinases and phosphatases, influencing various cellular functions simultaneously.
Conclusion: Second messengers are pivotal for the amplification and diversification of cellular signals. By activating specific downstream effectors, molecules like cAMP, IP₃, DAG, Ca²⁺, and cGMP enable cells to execute a wide array of functions in response to external stimuli. Understanding the roles and interactions of these second messengers is essential for elucidating the complexities of cellular communication and regulation.
4. How do feedback loops, both positive and negative, regulate signal transduction pathways to ensure appropriate cellular responses? Provide examples of each type of feedback in cell signaling.
Answer:
Feedback Loops in Signal Transduction: Feedback loops are regulatory mechanisms where the output of a process influences its own activity, either enhancing (positive feedback) or inhibiting (negative feedback) the process. They are essential for maintaining homeostasis, controlling the intensity and duration of signals, and ensuring precise cellular responses.
1. Negative Feedback:
- Definition: The output of a signaling pathway acts to inhibit its own production or activity, preventing overactivation and maintaining balance.
- Function: Maintains homeostasis by ensuring that signals do not become excessively strong or prolonged.
- Mechanism: Involves inhibitory proteins or pathways that counteract the initial signal.
Example: cAMP Pathway Regulation
- Scenario: Activation of GPCRs stimulates adenylyl cyclase to produce cAMP, which activates Protein Kinase A (PKA).
- Negative Feedback: PKA phosphorylates and activates phosphodiesterase (PDE), an enzyme that breaks down cAMP into AMP.
- Outcome: Reduces cAMP levels, thereby decreasing PKA activity and attenuating the signal.
Physiological Implications:
- Hormonal Regulation: In the endocrine system, negative feedback loops control hormone levels. For instance, high levels of thyroid hormones inhibit the release of thyrotropin-releasing hormone (TRH) and thyroid-stimulating hormone (TSH) from the pituitary gland, preventing excessive thyroid hormone production.
2. Positive Feedback:
- Definition: The output of a signaling pathway enhances its own production or activity, amplifying the signal.
- Function: Drives processes to completion, ensuring decisive and robust cellular responses.
- Mechanism: Involves activatory proteins or pathways that reinforce the initial signal.
Example: Blood Clotting Cascade
- Scenario: Injury to a blood vessel exposes collagen, activating platelets and initiating the clotting cascade.
- Positive Feedback: Thrombin generated in the cascade converts fibrinogen to fibrin and activates more platelets, which in turn produce more thrombin.
- Outcome: Rapid and extensive formation of a blood clot to prevent excessive bleeding.
Physiological Implications:
- Reproductive Processes: During ovulation, a surge in luteinizing hormone (LH) is triggered by positive feedback. High estrogen levels from the developing follicle lead to an LH surge, causing the release of the oocyte.
3. Balancing Positive and Negative Feedback:
Cells often utilize a combination of both feedback types to fine-tune responses. For instance, the initial positive feedback in the LH surge is eventually balanced by negative feedback mechanisms to prevent excessive hormone levels.
4. Integration in Signal Transduction Pathways:
Feedback loops are integrated within various signaling pathways to regulate signal duration, intensity, and specificity. They enable cells to respond dynamically to changing conditions and multiple simultaneous signals.
Conclusion: Feedback loops are fundamental for regulating signal transduction pathways, ensuring that cellular responses are appropriate, controlled, and sustainable. Negative feedback maintains equilibrium and prevents overstimulation, while positive feedback facilitates rapid and decisive actions. The interplay of these feedback mechanisms allows cells to effectively manage complex signaling environments and maintain physiological balance.
5. In what ways do scaffolding proteins contribute to the specificity and efficiency of signaling pathways, and what are the potential consequences of their dysfunction?
Answer:
Scaffolding Proteins in Signal Transduction: Scaffolding proteins are multifunctional proteins that organize and assemble specific signaling complexes by physically linking multiple components of a signaling pathway. They play a crucial role in enhancing the specificity, efficiency, and regulation of signal transduction.
Contributions to Specificity and Efficiency:
Spatial Organization:
- Localization of Signaling Components: Scaffolding proteins bring together kinases, phosphatases, and other signaling molecules in close proximity, ensuring that interactions occur only among designated partners.
- Example: KSR (Kinase Suppressor of Ras) scaffolds components of the MAPK/ERK pathway, facilitating efficient signal transmission from Raf to MEK to ERK.
Signal Amplification:
- Enhanced Interaction Rates: By tethering signaling molecules together, scaffolding proteins increase the likelihood of productive interactions, thereby amplifying the signal.
- Example: Ste5 in yeast organizes the kinases involved in the mating pheromone response, promoting robust activation of the MAPK cascade.
Prevention of Crosstalk:
- Pathway Segregation: Scaffolding proteins help segregate different signaling pathways, minimizing unintended interactions and maintaining pathway fidelity.
- Example: Axin scaffolds components of the Wnt signaling pathway, preventing interference from other pathways.
Temporal Coordination:
- Sequential Activation: Scaffolding proteins can coordinate the timing of signaling events, ensuring that each step of the pathway is activated in the correct order.
- Example: In the JNK signaling pathway, scaffold proteins ensure the timely activation of kinases, leading to precise regulation of gene expression.
Integration of Multiple Signals:
- Converging Inputs: Some scaffolding proteins can integrate signals from multiple receptors or pathways, allowing cells to coordinate complex responses.
- Example: Scaffold proteins in immune cells integrate signals from different receptors to modulate activation and response to pathogens.
Potential Consequences of Scaffolding Protein Dysfunction:
Loss of Signal Specificity:
- Increased Crosstalk: Without proper scaffolding, signaling molecules from different pathways may interact inappropriately, leading to mixed or unintended signals.
- Example: Disruption of KSR-mediated MAPK pathway assembly can result in aberrant activation of ERK by unrelated kinases.
Reduced Signal Efficiency:
- Inefficient Signaling: Absence or malfunction of scaffolding proteins can lead to decreased interaction rates among signaling components, weakening the overall signal.
- Example: Loss of Ste5 scaffolding in yeast impairs the pheromone response, reducing the cell’s ability to respond to mating signals.
Altered Signal Dynamics:
- Timing Disruptions: Malfunctioning scaffolding proteins can disrupt the temporal coordination of signaling events, leading to delayed or inappropriate cellular responses.
- Example: Impaired scaffolding in the Wnt pathway can affect the timing of β-catenin stabilization, altering gene expression patterns.
Disease Association:
- Cancer: Overactive scaffolding proteins can lead to persistent signaling pathways that drive uncontrolled cell proliferation and survival.
- Neurodegenerative Diseases: Impaired scaffolding in neuronal signaling pathways can disrupt synaptic function and neuronal health.
- Example: Mutations in axin scaffolding proteins are associated with colorectal cancer due to dysregulated Wnt signaling.
Developmental Defects:
- Impaired Tissue Formation: Scaffolding protein malfunctions can disrupt developmental signaling pathways, leading to congenital abnormalities and impaired tissue differentiation.
- Example: Defects in scaffold proteins involved in Hedgehog signaling can result in developmental disorders like holoprosencephaly.
Impaired Immune Responses:
- Dysregulated Signaling: Scaffolding protein malfunctions can affect immune cell activation and responses, compromising the immune system’s ability to fight infections.
- Example: Defective scaffolding in T-cell receptor signaling can impair T-cell activation and immune function.
Conclusion: Scaffolding proteins are essential for the precise and efficient operation of signal transduction pathways. By organizing and coordinating signaling complexes, they ensure that cellular responses are specific, robust, and appropriately regulated. Malfunctions in scaffolding proteins can lead to a wide range of biological dysfunctions and diseases, underscoring their critical role in maintaining cellular and organismal health. Understanding the roles of scaffolding proteins provides valuable insights into cellular signaling intricacies and offers potential targets for therapeutic interventions in various diseases.
6. How do receptor desensitization mechanisms function in G-Protein Coupled Receptor (GPCR) signaling, and what are the physiological consequences of impaired desensitization?
Answer:
Receptor Desensitization in GPCR Signaling: Receptor desensitization is a regulatory process that reduces the responsiveness of G-Protein Coupled Receptors (GPCRs) to continuous or repeated stimulation by ligands. This mechanism prevents overstimulation, maintains cellular homeostasis, and ensures that cells can respond appropriately to fluctuating signal strengths.
Mechanisms of Receptor Desensitization:
Phosphorylation by GPCR Kinases (GRKs):
- Role of GRKs: GRKs specifically phosphorylate activated GPCRs on serine and threonine residues within the receptor’s cytoplasmic domains.
- Effect of Phosphorylation: Phosphorylation decreases the receptor’s affinity for G-proteins, reducing its ability to activate downstream signaling pathways.
Binding of Arrestin Proteins:
- Function of Arrestins: Arrestins (e.g., β-arrestin) bind to phosphorylated GPCRs, blocking further G-protein coupling.
- Additional Roles of Arrestins:
- Receptor Internalization: Arrestin-bound receptors are targeted for internalization via clathrin-coated pits, removing them from the cell surface.
- Alternative Signaling: Arrestins can initiate alternative signaling pathways independent of G-proteins, contributing to diverse cellular responses.
Receptor Internalization and Downregulation:
- Endocytosis: Activated and phosphorylated GPCRs are internalized into the cell through endocytosis.
- Recycling vs. Degradation:
- Recycling: Receptors can be dephosphorylated and returned to the plasma membrane for reuse.
- Degradation: Receptors can be directed to lysosomes for degradation, reducing receptor numbers on the cell surface.
Regulation by Ubiquitination:
- Ubiquitin Ligases: Certain ubiquitin ligases tag desensitized receptors for degradation.
- Impact: Enhances receptor downregulation and prevents reactivation without new receptor synthesis.
Physiological Consequences of Impaired Desensitization:
Chronic Overstimulation:
- Persistent Signaling: Impaired desensitization leads to prolonged activation of GPCRs, causing continuous downstream signaling.
- Example: Chronic exposure to high levels of β-adrenergic agonists can result in excessive heart rate and contractility, potentially leading to cardiac hypertrophy.
Receptor Downregulation Defects:
- Receptor Overexpression: Failure to internalize and degrade desensitized receptors can result in receptor overexpression, enhancing sensitivity to ligands.
- Example: Overactive GPCR signaling is implicated in certain cancers, where receptors like β2-adrenergic receptors may remain active, promoting tumor growth and survival.
Tolerance Development:
- Pharmacological Tolerance: Impaired desensitization can prevent the development of tolerance to drugs targeting GPCRs, necessitating higher doses for therapeutic effects.
- Example: In asthma treatment, inadequate desensitization of β2-adrenergic receptors can lead to reduced drug efficacy over time.
Immune System Dysregulation:
- Excessive Immune Responses: GPCR desensitization plays a role in regulating immune cell responses. Impaired desensitization can result in hyperactive immune cells, contributing to autoimmune diseases.
- Example: Overactive chemokine receptors on T cells can lead to excessive migration and inflammation in autoimmune conditions.
Neurological Disorders:
- Imbalanced Neurotransmission: GPCRs are critical in neurotransmitter signaling. Impaired desensitization can disrupt synaptic plasticity, affecting learning, memory, and mood regulation.
- Example: Dysregulated dopamine receptor desensitization is associated with psychiatric disorders like schizophrenia and addiction.
Cardiovascular Diseases:
- Hypertension and Heart Failure: Impaired desensitization of GPCRs involved in vascular tone regulation can contribute to hypertension and heart failure due to uncontrolled vasoconstriction and cardiac stress.
- Example: Persistent activation of angiotensin II receptors can lead to vasoconstriction, increased blood pressure, and adverse cardiac remodeling.
Conclusion: Receptor desensitization is a vital regulatory mechanism in GPCR signaling that ensures cells respond appropriately to varying levels of stimuli. Impaired desensitization disrupts this balance, leading to excessive or prolonged signaling that can contribute to a range of physiological and pathological conditions, including cardiovascular diseases, cancer, immune disorders, and neurological issues. Understanding desensitization mechanisms is essential for developing therapeutic strategies that target GPCRs effectively while minimizing adverse effects.
7. How do cells integrate multiple signaling pathways to produce a coordinated response, and what factors influence the outcome of such integration?
Answer:
Integration of Multiple Signaling Pathways: Cells often receive and process multiple signals simultaneously, necessitating the integration of diverse signaling pathways to generate coherent and appropriate responses. This integration allows cells to respond dynamically to complex and changing environments, ensuring precise regulation of cellular functions.
Mechanisms of Signal Integration:
Convergence Points:
- Shared Downstream Effectors: Different signaling pathways may converge on common downstream molecules, enabling coordinated regulation.
- Example: Both the MAPK/ERK and PI3K/Akt pathways can influence gene expression through the activation of transcription factors like CREB.
Divergence Points:
- Branching Pathways: A single signaling molecule can activate multiple downstream pathways, leading to diverse cellular outcomes.
- Example: Activation of RTKs can simultaneously trigger the MAPK/ERK pathway for cell proliferation and the PI3K/Akt pathway for cell survival.
Cross-Talk Between Pathways:
- Direct Interactions: Components of one pathway can directly interact with components of another, modulating each other’s activity.
- Indirect Interactions: Pathways can influence each other through intermediary proteins or feedback loops.
- Example: The Wnt signaling pathway can interact with the PI3K/Akt pathway to regulate cell proliferation and survival.
Scaffold Proteins:
- Organizing Signaling Complexes: Scaffold proteins bring together multiple signaling molecules, enhancing specificity and efficiency.
- Example: KSR (Kinase Suppressor of Ras) scaffolds components of the MAPK/ERK pathway, ensuring efficient signal transmission and preventing crosstalk with unrelated pathways.
Temporal Dynamics:
- Sequential Activation: Signaling pathways can be activated in a specific temporal order, allowing one pathway to influence the activation of another.
- Example: Initial activation of a kinase cascade can prime cells to respond to subsequent signals by modifying transcription factors.
Spatial Localization:
- Compartmentalization: Signaling components localized to specific cellular regions (e.g., lipid rafts, endosomes) can selectively interact with certain pathways.
- Example: Signals initiated at the plasma membrane may differ from those initiated from endosomal compartments, leading to distinct outcomes.
Factors Influencing Signal Integration Outcomes:
Signal Strength and Duration:
- Intensity: The strength of each signal can determine the extent of pathway activation and the resultant cellular response.
- Duration: Prolonged versus transient signals can lead to different outcomes, such as sustained gene expression versus temporary metabolic changes.
Receptor Density and Distribution:
- Availability: The number and distribution of receptors on the cell surface influence the cell’s responsiveness to different signals.
- Example: High density of growth factor receptors may enhance sensitivity to mitogenic signals, promoting cell proliferation.
Scaffold and Adaptor Proteins:
- Organization of Signaling Complexes: Scaffold proteins facilitate specific interactions, ensuring that only relevant pathways are activated.
- Example: Axin scaffolds components of the Wnt signaling pathway, preventing interference from other pathways.
Feedback Mechanisms:
- Regulation of Pathway Activity: Positive and negative feedback loops can modulate the activity and integration of signaling pathways.
- Example: Negative feedback in the MAPK pathway can limit signal duration, allowing other pathways to influence the final response.
Post-Translational Modifications:
- Protein Modifications: Phosphorylation, ubiquitination, and other modifications can alter the activity, localization, or interactions of signaling proteins, affecting pathway integration.
- Example: Phosphorylation of a transcription factor by one pathway can influence its ability to be activated by another pathway.
Cellular Context and State:
- Differentiation Status: Stem cells versus differentiated cells may respond differently to the same signals due to variations in signaling machinery.
- Metabolic State: Nutrient availability and energy status can influence how signals are interpreted and integrated.
Competition for Signaling Components:
- Resource Allocation: Multiple pathways may compete for the same signaling molecules, affecting the overall outcome based on pathway priority and availability.
- Example: Competing activation of Raf by different upstream signals can influence the strength and specificity of the MAPK response.
Examples of Signal Integration:
Cell Cycle Regulation:
- Growth Factor and DNA Damage Signals: Integration of mitogenic signals (e.g., via RTKs) promoting cell cycle progression and stress signals (e.g., via p53) inducing cell cycle arrest or apoptosis.
- Outcome: Decision to proceed with division, repair DNA, or undergo apoptosis based on the balance of signals.
Immune Cell Activation:
- Antigen Recognition and Co-Stimulation: T cells integrate signals from the T cell receptor (antigen recognition) and co-stimulatory receptors (e.g., CD28) to fully activate immune responses.
- Outcome: Activation of T cells leads to proliferation, cytokine production, and differentiation into effector cells.
Neuronal Plasticity:
- Synaptic Activity and Growth Factors: Neurons integrate electrical signals from synaptic activity with growth factor signals to regulate synaptic strength and dendritic spine formation.
- Outcome: Modulation of synaptic connections underlying learning and memory.
Conclusion: Cells employ intricate mechanisms to integrate multiple signaling pathways, ensuring that responses are precise, coordinated, and appropriate to the cellular context. Factors such as signal strength, temporal dynamics, spatial localization, and the presence of scaffolding proteins play pivotal roles in determining the outcome of signal integration. Understanding how cells integrate signals is fundamental to elucidating complex biological processes and addressing dysregulated signaling in diseases.
8. What mechanisms do cells employ to maintain the fidelity of signal transduction pathways, preventing erroneous or excessive cellular responses?
Answer:
Maintaining Fidelity in Signal Transduction: Signal transduction pathways must operate with high fidelity to ensure accurate and appropriate cellular responses. Cells employ multiple mechanisms to maintain this precision, preventing erroneous or excessive activation that could lead to dysfunction or disease.
Key Mechanisms Ensuring Signal Fidelity:
Specificity of Receptors and Ligands:
- High Affinity Interactions: Receptors have specific binding sites that recognize particular ligands, ensuring that only appropriate signals are transmitted.
- Structural Complementarity: The three-dimensional structure of receptors and ligands ensures precise binding, reducing cross-reactivity.
- Example: Insulin receptors specifically bind insulin, initiating distinct signaling pathways related to glucose uptake.
Signal Amplification Control:
- Regulated Cascade Steps: Each step in a signaling cascade is tightly controlled, ensuring that amplification does not lead to excessive signal output.
- Feedback Inhibition: Negative feedback loops dampen signal amplification once a threshold is reached.
- Example: In the MAPK/ERK pathway, activated ERK can phosphorylate upstream kinases to inhibit further pathway activation, preventing overamplification.
Scaffold Proteins and Spatial Compartmentalization:
- Organizing Signaling Complexes: Scaffold proteins ensure that signaling molecules interact only within specific complexes, maintaining pathway specificity.
- Localized Signaling: Compartmentalization of signaling components to specific cellular regions (e.g., lipid rafts, endosomes) restricts signal propagation to intended targets.
- Example: Axin scaffolds the Wnt signaling components, preventing crosstalk with other pathways.
Temporal Regulation:
- Timed Activation and Termination: Signals are activated and terminated in a timely manner to ensure transient responses rather than prolonged activation.
- Kinetic Control: The rates of activation and deactivation of signaling molecules are finely tuned.
- Example: Phosphatases rapidly dephosphorylate kinases in the MAPK pathway, ensuring that ERK activation is transient.
Negative Feedback Loops:
- Inhibition of Upstream Signals: Activated signaling components induce the expression or activation of inhibitors that suppress further pathway activation.
- Example: Activated PKA in the cAMP pathway phosphorylates and activates phosphodiesterase (PDE), which degrades cAMP, reducing PKA activity.
Post-Translational Modifications:
- Phosphorylation and Dephosphorylation: Dynamic addition and removal of phosphate groups regulate the activity of signaling proteins.
- Ubiquitination: Tags proteins for degradation, ensuring that signaling components do not accumulate excessively.
- Example: Ubiquitin ligases target misfolded or excessive signaling proteins for proteasomal degradation, preventing aberrant signaling.
Signal Termination Mechanisms:
- Ligand Degradation: Enzymes degrade signaling molecules, reducing their availability to receptors.
- Receptor Internalization: Endocytosis removes receptors from the cell surface, terminating signal transduction.
- Example: EGF is internalized and degraded after binding to its receptor, terminating the EGF signaling pathway.
Protein Scaffolding and Localization:
- Scaffold Proteins: Organize signaling complexes to facilitate specific interactions and prevent unintended cross-pathway interactions.
- Subcellular Localization: Ensures that signaling molecules are in the correct cellular compartment to interact with their intended partners.
- Example: Ste5 scaffolds MAPK components in yeast, ensuring specific activation of the pheromone response pathway.
Redundancy and Robustness:
- Multiple Pathways for the Same Response: Ensures that if one pathway is disrupted, others can compensate, maintaining overall signal fidelity.
- Robust Feedback Controls: Multiple layers of regulation prevent runaway signaling.
- Example: Multiple phosphatases regulate the MAPK pathway, providing redundancy in signal termination.
Consequences of Compromised Signal Fidelity:
- Uncontrolled Cell Proliferation: Loss of negative feedback can lead to excessive cell growth, contributing to cancer.
- Impaired Development: Erroneous signaling can disrupt developmental processes, leading to congenital abnormalities.
- Neurodegenerative Diseases: Faulty signal termination can cause neuronal dysfunction and death.
- Metabolic Disorders: Dysregulated signaling pathways can result in conditions like diabetes or obesity.
Conclusion: Cells utilize a multifaceted array of mechanisms to maintain the fidelity of signal transduction pathways. Through specificity of interactions, controlled amplification, spatial and temporal regulation, and robust feedback systems, cells ensure accurate and appropriate responses to diverse stimuli. Disruptions in these regulatory mechanisms can lead to a variety of diseases, highlighting the importance of precise control in cellular signaling processes.
9. What are the roles of cell junctions in cell communication, and how do different types of junctions contribute to tissue integrity and function?
Answer:
Cell Junctions in Cell Communication: Cell junctions are specialized structures that connect adjacent cells, facilitating communication and maintaining tissue integrity. They play critical roles in coordinating cellular activities, regulating the passage of substances between cells, and providing mechanical support to tissues.
Types of Cell Junctions and Their Functions:
Tight Junctions (Occluding Junctions):
- Structure: Composed of claudin and occludin proteins that form a seal between adjacent cells near their apical surfaces.
- Function:
- Barrier Formation: Prevent the free passage of molecules and ions through the space between cells, maintaining distinct extracellular environments.
- Apical-Basal Polarity: Help establish and maintain the polarity of epithelial cells by preventing the mixing of apical and basolateral membrane proteins.
- Location: Predominantly found in epithelial tissues lining organs and body cavities (e.g., intestinal epithelium, blood-brain barrier).
Adherens Junctions (Zonula Adherens):
- Structure: Comprised of cadherin proteins linked to the actin cytoskeleton via catenins.
- Function:
- Mechanical Attachment: Provide strong mechanical bonds between cells, maintaining tissue integrity.
- Signal Transduction: Involved in transmitting signals that regulate cell behavior and differentiation.
- Location: Common in epithelial and endothelial tissues, forming a belt-like structure encircling the cell.
Desmosomes (Macula Adherens):
- Structure: Consist of cadherins (desmogleins and desmocollins) linked to intermediate filaments (keratin) via adaptor proteins (desmoplakins and plakoglobins).
- Function:
- Mechanical Strength: Distribute tensile forces across tissues, preventing cells from tearing apart under stress.
- Tissue Integrity: Essential for maintaining the structural integrity of tissues subjected to mechanical stress, such as skin and cardiac muscle.
- Location: Abundant in tissues like the epidermis, heart muscle, and uterus.
Gap Junctions:
- Structure: Composed of connexin proteins that form channels (connexons) allowing direct cytoplasmic communication between adjacent cells.
- Function:
- Electrical and Chemical Coupling: Permit the passage of ions, metabolites, and signaling molecules, enabling coordinated cellular activities.
- Synchronization: Essential for synchronized muscle contractions and coordinated responses in neuronal networks.
- Location: Found in cardiac muscle, smooth muscle, neurons, and various epithelial tissues.
Hemidesmosomes:
- Structure: Similar to desmosomes but connect cells to the extracellular matrix instead of adjacent cells. Composed of integrins linked to intermediate filaments.
- Function:
- Anchoring: Secure epithelial cells to the underlying basement membrane, providing stability and resistance to shear stress.
- Location: Prominently present in epithelial tissues, such as the skin’s basal layer.
Anchoring Junctions:
- Includes: Desmosomes and hemidesmosomes.
- Function: Provide strong adhesion between cells and between cells and the extracellular matrix, ensuring tissue resilience.
Contribution to Tissue Structure and Function:
Mechanical Stability:
- Tissue Cohesion: Desmosomes and adherens junctions distribute mechanical forces across tissues, preventing cells from detaching or being damaged under stress.
- Structural Support: Gap junctions and tight junctions help maintain the organized structure of tissues by ensuring cells remain connected and aligned.
Barrier Function:
- Selective Permeability: Tight junctions regulate the movement of substances between cells, maintaining distinct environments on either side of epithelial layers (e.g., preventing leakage in the blood-brain barrier).
- Protection: By forming barriers, cell junctions protect underlying tissues from pathogens and harmful substances.
Cell Communication:
- Direct Cytoplasmic Exchange: Gap junctions allow cells to share ions and small molecules, facilitating coordinated responses and metabolic cooperation.
- Signal Transduction: Adherens junctions participate in signaling pathways that influence cell proliferation, differentiation, and migration.
Cellular Organization:
- Polarity Maintenance: Tight junctions help establish and preserve the polarity of epithelial cells, ensuring directional transport of materials (e.g., nutrient absorption in the intestines).
- Compartmentalization: Junctions contribute to the spatial organization of cells within tissues, essential for specialized functions.
Consequences of Cell Junction Dysfunction:
Loss of Tissue Integrity:
- Detachment and Fragility: Impaired desmosomes or hemidesmosomes can lead to weakened tissue cohesion, making tissues susceptible to injury and detachment (e.g., blistering skin diseases like pemphigus vulgaris).
Barrier Dysfunction:
- Leakage and Inflammation: Disrupted tight junctions can cause leaky barriers, allowing pathogens and toxins to enter tissues and trigger inflammatory responses (e.g., leaky gut syndrome).
Impaired Communication:
- Uncoordinated Activities: Defective gap junctions can lead to desynchronized cellular activities, affecting processes like heart contractions and neural signaling.
Cancer Progression:
- Metastasis: Loss of cell adhesion properties can facilitate cancer cell detachment and spread to other parts of the body.
- Tumor Growth: Impaired signaling through adherens junctions can contribute to uncontrolled cell proliferation.
Conclusion: Cell junctions are fundamental to maintaining the structural integrity, selective permeability, and coordinated function of tissues in animal cells. By providing mechanical stability, regulating substance movement, and facilitating communication, they ensure the proper organization and operation of multicellular organisms. Dysfunction in cell junctions can lead to a range of diseases, highlighting their critical role in health and disease.
10. How do lysosomes contribute to cellular waste management, and what are the consequences of lysosomal dysfunction in human health?
Answer:
Lysosomes in Cellular Waste Management: Lysosomes are membrane-bound organelles found in eukaryotic cells that function as the cell’s waste disposal system. They contain a variety of hydrolytic enzymes capable of breaking down all types of biological polymers, including proteins, nucleic acids, carbohydrates, and lipids. Lysosomes play a crucial role in degrading and recycling cellular waste, damaged organelles, and engulfed pathogens.
Functions of Lysosomes:
Intracellular Digestion:
- Autophagy: Lysosomes digest and recycle damaged or obsolete organelles and macromolecules within the cell. Autophagosomes engulf the targeted components and fuse with lysosomes, where the contents are broken down and recycled.
- Endocytosis: Lysosomes digest materials ingested from the extracellular environment via endocytosis. Phagosomes (from phagocytosis) and endosomes (from pinocytosis) fuse with lysosomes to degrade their contents.
Waste Removal:
- Breakdown of Cellular Debris: Lysosomes degrade cellular waste products, preventing accumulation of harmful substances and maintaining cellular homeostasis.
Defense Mechanism:
- Pathogen Destruction: In immune cells like macrophages and neutrophils, lysosomes digest engulfed pathogens such as bacteria and viruses, contributing to the body’s defense against infections.
Apoptosis (Programmed Cell Death):
- Caspase Activation: Lysosomal enzymes can be released into the cytoplasm to activate caspases, enzymes that execute apoptosis, aiding in controlled cell death.
Recycling of Cellular Components:
- Reuse of Molecules: Digested materials are transported out of lysosomes and reused for various cellular processes, conserving resources and energy.
Consequences of Lysosomal Dysfunction:
Lysosomal Storage Diseases:
- Definition: Genetic disorders caused by deficiencies in specific lysosomal enzymes, leading to the accumulation of undigested substrates.
- Examples:
- Gaucher Disease: Deficiency in glucocerebrosidase leads to the buildup of glucocerebroside in macrophages.
- Tay-Sachs Disease: Deficiency in hexosaminidase A results in the accumulation of GM2 gangliosides in neurons.
- Pompe Disease: Deficiency in acid alpha-glucosidase causes glycogen accumulation in muscle cells.
- Consequences: Accumulation of substrates disrupts cellular function, leading to organ enlargement, neurological symptoms, and impaired development.
Cellular Damage and Death:
- Accumulation of Waste: Undigested substrates can interfere with cellular processes, causing organelle dysfunction and impaired metabolism.
- Autophagic Blockage: Incomplete autophagy results in the buildup of damaged organelles, contributing to cellular stress and apoptosis.
- Apoptosis Induction: Excessive release of lysosomal enzymes into the cytoplasm can trigger uncontrolled cell death, impacting tissue integrity and function.
Neurodegenerative Diseases:
- Alzheimer’s Disease: Impaired lysosomal function is associated with the accumulation of amyloid-beta plaques and tau tangles.
- Parkinson’s Disease: Dysfunctional lysosomes contribute to the buildup of alpha-synuclein aggregates, affecting neuronal health.
Immune System Impairment:
- Reduced Pathogen Clearance: Defective lysosomes in immune cells hinder the digestion of engulfed pathogens, compromising the body’s ability to fight infections.
Cancer Progression:
- Tumorigenesis: Lysosomal dysfunction can contribute to cancer progression by affecting cellular signaling pathways, apoptosis, and autophagy, enabling cancer cells to evade death and sustain growth.
Mechanisms Preventing Lysosomal Dysfunction:
Proper Enzyme Targeting:
- Mannose-6-Phosphate Tagging: Lysosomal enzymes are tagged with mannose-6-phosphate in the Golgi apparatus, ensuring their delivery to lysosomes via mannose-6-phosphate receptors.
Quality Control Systems:
- ER-Associated Degradation (ERAD): Misfolded lysosomal enzymes are identified and targeted for degradation before they reach lysosomes.
- Chaperone Proteins: Assist in proper folding of lysosomal enzymes, preventing accumulation of non-functional proteins.
Autophagy Regulation:
- Efficient Autophagic Flux: Ensures timely digestion and recycling of cellular components, maintaining lysosomal health and function.
Conclusion: Lysosomes are essential for cellular waste management, recycling, and defense mechanisms. Their dysfunction can lead to severe cellular and systemic consequences, including lysosomal storage diseases, neurodegeneration, immune impairment, and cancer progression. Understanding lysosomal biology is crucial for diagnosing and developing treatments for these conditions, highlighting the organelles’ pivotal role in maintaining cellular and organismal health.
11. How do cells communicate through direct contact, and what are some key examples of processes that utilize contact-dependent signaling?
Answer:
Direct Contact Communication Overview: Cells can communicate through direct physical interactions, requiring cell-to-cell contact. This mode of communication is essential for maintaining tissue integrity, coordinating cellular activities, and regulating developmental processes. Contact-dependent signaling involves membrane-bound ligands and receptors, allowing precise and localized signal transmission.
Mechanisms of Contact-Dependent Signaling:
Juxtacrine Signaling:
- Definition: A form of cell signaling where the signal-emitting and signal-receiving cells are in direct contact.
- Components: Membrane-bound ligands on one cell interact with membrane-bound receptors on an adjacent cell.
- Types:
- Signaling through Direct Membrane Proteins: Ligands and receptors remain attached to the membranes of their respective cells during signaling.
- Gap Junction Communication: Direct cytoplasmic connections between cells allow the passage of ions and small molecules.
Contact-Mediated Induction:
- Definition: A process where cell fate is determined through direct contact with neighboring cells.
- Function: Critical in embryonic development and tissue differentiation, ensuring that cells develop specific identities based on their position and interactions.
Neural Synapses:
- Definition: Specialized junctions where neurons communicate with other neurons, muscle cells, or glands.
- Mechanism: Electrical or chemical synapses facilitate rapid and specific signal transmission across the synaptic cleft.
Immunological Synapses:
- Definition: Structured interfaces between T cells and antigen-presenting cells (APCs).
- Function: Enable precise recognition of antigens and activation of immune responses through the clustering of receptors and signaling molecules.
Key Examples of Processes Utilizing Contact-Dependent Signaling:
Developmental Patterning:
- Notch Signaling Pathway:
- Mechanism: Interaction between Notch receptors on one cell and Delta/Jagged ligands on an adjacent cell.
- Function: Influences cell differentiation, proliferation, and apoptosis during embryonic development.
- Example: Determination of neuronal and glial cell fate in the nervous system.
- Notch Signaling Pathway:
Immune Cell Activation:
- T-Cell Activation:
- Mechanism: T cell receptors (TCRs) on T cells recognize antigens presented by MHC molecules on APCs.
- Function: Triggers T cell activation, proliferation, and differentiation into effector cells.
- Example: Activation of helper T cells by dendritic cells presenting foreign antigens.
- T-Cell Activation:
Cell Adhesion and Tissue Formation:
- Cadherin-Mediated Adhesion:
- Mechanism: Cadherin proteins on adjacent cells interact through homophilic binding, linking cells together.
- Function: Maintains tissue structure and integrity by mediating cell-cell adhesion.
- Example: Formation and maintenance of epithelial layers through E-cadherin interactions.
- Cadherin-Mediated Adhesion:
Muscle Contraction:
- Neuromuscular Junctions:
- Mechanism: Motor neurons form synapses with muscle fibers, releasing neurotransmitters like acetylcholine.
- Function: Induces muscle fiber depolarization and contraction.
- Example: Rapid communication between motor neurons and skeletal muscle fibers to facilitate movement.
- Neuromuscular Junctions:
Stem Cell Niche Maintenance:
- Hedgehog Signaling in Stem Cell Niches:
- Mechanism: Hedgehog ligands are presented on niche cells and interact with receptors on stem cells.
- Function: Regulates stem cell maintenance, self-renewal, and differentiation.
- Example: Maintenance of hematopoietic stem cells in the bone marrow niche.
- Hedgehog Signaling in Stem Cell Niches:
Apoptosis Induction:
- Fas-Fas Ligand Interaction:
- Mechanism: Fas ligand (FasL) on one cell binds to Fas receptor on another cell.
- Function: Triggers apoptosis in the Fas-expressing cell, important for immune regulation and eliminating damaged cells.
- Example: Induction of apoptosis in infected or cancerous cells by cytotoxic T lymphocytes.
- Fas-Fas Ligand Interaction:
Synaptic Plasticity:
- Neuronal Synapse Formation and Remodeling:
- Mechanism: Neurons form synapses through direct contact, allowing the establishment and modification of neural networks.
- Function: Essential for learning, memory, and adaptation of the nervous system.
- Example: Formation of new synaptic connections in response to learning stimuli.
- Neuronal Synapse Formation and Remodeling:
Conclusion: Contact-dependent signaling is a fundamental mode of cellular communication that ensures precise and coordinated responses in multicellular organisms. Through direct interactions between membrane-bound ligands and receptors, cells can regulate a wide array of processes, including development, immune responses, tissue integrity, and specialized functions like muscle contraction and neuronal communication. Understanding these mechanisms is crucial for comprehending how complex tissues and organs are organized and maintained.
12. How do lysosomes and peroxisomes differ in their functions and contributions to cellular metabolism, and what are the implications of their dysfunction in human diseases?
Answer:
Lysosomes and Peroxisomes in Cellular Metabolism: Lysosomes and peroxisomes are both membrane-bound organelles involved in cellular metabolism, but they perform distinct functions and contribute differently to cellular homeostasis.
Lysosomes:
Function:
- Intracellular Digestion: Lysosomes contain a variety of hydrolytic enzymes that break down all types of biological polymers, including proteins, nucleic acids, carbohydrates, and lipids.
- Autophagy: Lysosomes digest and recycle damaged or obsolete organelles and macromolecules within the cell.
- Endocytosis: Lysosomes degrade materials ingested from the extracellular environment via endocytosis.
- Defense Mechanism: In immune cells like macrophages and neutrophils, lysosomes digest engulfed pathogens.
Contribution to Cellular Metabolism:
- Waste Removal: Prevents accumulation of cellular waste products, maintaining cellular homeostasis.
- Recycling: Provides building blocks for new cellular components by breaking down macromolecules.
- Energy Production: Indirectly contributes by recycling nutrients that can be used in metabolic pathways.
Implications of Lysosomal Dysfunction:
- Lysosomal Storage Diseases: Genetic deficiencies in specific lysosomal enzymes lead to accumulation of undigested substrates, causing cellular and tissue dysfunction.
- Examples: Gaucher disease, Tay-Sachs disease, Pompe disease.
- Neurodegenerative Diseases: Impaired lysosomal function is associated with accumulation of toxic proteins in diseases like Alzheimer’s and Parkinson’s.
- Immune Impairment: Defective lysosomes reduce the ability of immune cells to clear pathogens, increasing susceptibility to infections.
- Lysosomal Storage Diseases: Genetic deficiencies in specific lysosomal enzymes lead to accumulation of undigested substrates, causing cellular and tissue dysfunction.
Peroxisomes:
Function:
- Fatty Acid Metabolism: Peroxisomes are involved in the β-oxidation of very-long-chain fatty acids, converting them into medium-chain fatty acids that can be further processed in mitochondria.
- Reactive Oxygen Species (ROS) Detoxification: Contain enzymes like catalase that break down hydrogen peroxide (H₂O₂), a reactive oxygen species, into water and oxygen.
- Bile Acid Synthesis: Play a role in the synthesis of bile acids from cholesterol.
- Detoxification of Harmful Substances: Metabolize toxic compounds, including ethanol and fatty alcohols.
Contribution to Cellular Metabolism:
- Lipid Metabolism: Essential for processing fatty acids and maintaining lipid homeostasis.
- Detoxification: Protects cells from oxidative damage by detoxifying reactive oxygen species and harmful substances.
- Biochemical Pathways: Participate in biosynthetic pathways necessary for cellular functions and signaling.
Implications of Peroxisomal Dysfunction:
- Peroxisomal Disorders: Genetic mutations affecting peroxisomal enzymes lead to metabolic dysfunctions.
- Examples: Zellweger syndrome, Adrenoleukodystrophy, Refsum disease.
- Lipid Accumulation: Defects in fatty acid metabolism result in the accumulation of very-long-chain fatty acids, which can disrupt cellular membranes and organelle function.
- Oxidative Stress: Impaired ROS detoxification leads to increased oxidative damage, contributing to aging and various diseases.
- Neurological Impairment: Accumulation of toxic lipids and oxidative damage in the nervous system can cause severe neurological deficits and developmental delays.
- Peroxisomal Disorders: Genetic mutations affecting peroxisomal enzymes lead to metabolic dysfunctions.
Differences Between Lysosomes and Peroxisomes:
Feature | Lysosomes | Peroxisomes |
---|---|---|
Primary Function | Intracellular digestion and recycling | Fatty acid metabolism and ROS detoxification |
Enzyme Content | Hydrolytic enzymes (proteases, lipases, nucleases) | Oxidative enzymes (catalase, acyl-CoA oxidases) |
Energy Metabolism | Indirect role through recycling | Direct role in lipid metabolism and energy production |
Reactive Oxygen Species | Limited detoxification via enzymes like catalase | Extensive detoxification of H₂O₂ and other ROS |
Biogenesis | Formed from the Golgi apparatus | Bud from the endoplasmic reticulum |
Implications of Dysfunction | Lysosomal storage diseases, neurodegeneration | Peroxisomal disorders, lipid accumulation, oxidative stress |
Conclusion: Lysosomes and peroxisomes are vital organelles that contribute uniquely to cellular metabolism and homeostasis. Lysosomes focus on waste degradation and recycling, while peroxisomes handle lipid metabolism and detoxification of reactive oxygen species. Dysfunction in either organelle can lead to severe metabolic and neurological diseases, highlighting their essential roles in maintaining cellular and organismal health. Understanding their distinct functions and regulatory mechanisms is crucial for diagnosing and developing treatments for related disorders.
Conclusion: These twelve thought-provoking questions and detailed answers cover essential aspects of cell communication, including types of signaling, receptor functions, second messengers, feedback loops, scaffolding proteins, receptor desensitization, signal integration, cell junctions, and organelle functions related to signaling pathways. They are designed to deepen understanding, promote critical thinking, and reinforce key concepts essential for mastering cell biology. Utilizing these questions as study aids can help learners grasp the complexities of cellular communication and the mechanisms that ensure precise and coordinated cellular responses.