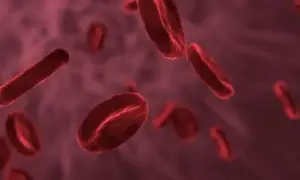
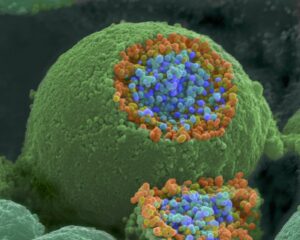
Introduction to Cell Biology
Cell biology is the cornerstone of understanding life at its most fundamental level. It provides insights into how organisms grow, develop, and function by examining the structure, behavior, and processes within individual cells. As a vital branch of science, it builds the conceptual framework for topics across biology, including genetics, physiology, and ecology. At the heart of this field lies an in-depth study of cell structure, which reveals the specialized components—organelles, membranes, and cytoskeletal elements—that enable cells to carry out life-sustaining functions.
Cell biology also explores dynamic activities within cells, including cell physiology, the complex biochemical reactions and signaling mechanisms that govern metabolism, growth, and response to stimuli. In understanding how cells divide and reproduce, students investigate the cell cycle, while studies in cell development help clarify how single-celled organisms give rise to multicellular complexity. The ability of cells to coordinate their actions through cell communication is crucial for maintaining tissue function and orchestrating responses across systems.
Because cell biology intersects closely with genetics, it provides the foundation for understanding gene expression and regulation. Within the domain of molecular genetics, students explore how DNA and RNA store and transmit information, and how protein synthesis transforms genetic instructions into cellular machinery. Related topics such as gene expression, genetic mutation, and the molecular basis of inheritance are essential for understanding how traits are passed on and how diseases arise at the cellular level.
The applications of cell biology extend to modern medical and biotechnological advancements. Topics such as genetic applications in medicine and DNA technology have revolutionized diagnostics, therapeutics, and personalized treatment. Students also gain experience with molecular techniques in research, which empower discoveries in everything from cancer biology to regenerative medicine.
On a broader scale, the knowledge of cellular function connects with population-level phenomena such as population genetics and quantitative genetics. It also informs understanding of species adaptation through molecular evolution and genome-wide changes as explored in genomics. Foundations in Mendelian genetics remain essential for bridging classic and modern approaches. Beyond genetics, cell biology contributes to understanding larger biological contexts, such as environmental interactions studied in ecology and long-term changes explained by evolutionary biology.
In short, cell biology is the gateway to understanding life from the inside out. Its principles illuminate how living organisms develop, adapt, heal, and evolve, making it a foundational subject for students entering biological and biomedical sciences.
Table of Contents
Five Main Sub-Areas of Cell Biology
Cell biology encompasses various specialized sub-areas, each focusing on different aspects of cells and their functions. Here are five main sub-areas:
Cell Structure and Ultrastructure
- This sub-area focuses on understanding the physical components of cells, including their shapes, sizes, and organization.
- Key topics include:
- The study of cell organelles such as the nucleus, mitochondria, chloroplasts, Golgi apparatus, and endoplasmic reticulum.
- Analysis of the cytoskeleton (microtubules, actin filaments) that provides shape and facilitates intracellular transport.
- Techniques such as electron microscopy are commonly used to examine the ultrastructure of cells at high resolution.
Cell Physiology and Biochemistry
- This area examines the biochemical and physiological processes within cells that are essential for their survival and function.
- Key topics include:
- Metabolic pathways, such as glycolysis, the Krebs cycle, and oxidative phosphorylation.
- Processes like protein synthesis, enzyme activity, and signal transduction pathways.
- Understanding how cells produce and manage energy, such as ATP production in mitochondria.
- Foundational knowledge in this sub-area is vital in biomedical research and pharmacology. For example, research supported by institutions like the journal Trends in Biochemical Sciences highlights ongoing discoveries in cell metabolism and regulation.
Cell Communication and Signaling
- Focuses on how cells interact with their environment and communicate with each other to coordinate activities.
- Key topics include:
- Signal transduction pathways that mediate responses to hormones, growth factors, and other signaling molecules.
- Cell membrane receptors (e.g., G-protein coupled receptors, ion channels) and their roles in cellular responses.
- Mechanisms of cell-to-cell communication, such as gap junctions, neurotransmitter release, and immune cell signaling.
Cell Cycle and Division
- This sub-area investigates how cells grow, replicate their DNA, and divide to produce new cells.
- Key topics include:
- The stages of the cell cycle: G1, S, G2, and M (mitosis or meiosis).
- The regulation of the cell cycle by proteins like cyclins and cyclin-dependent kinases (CDKs).
- Processes of mitosis (for growth and repair) and meiosis (for sexual reproduction).
- Study of abnormalities in the cell cycle, such as those leading to cancer.
Cell Development and Differentiation
- Examines how cells specialize and change during development to form different tissues and organs.
- Key topics include:
- Mechanisms of stem cell differentiation into specific cell types (e.g., nerve cells, muscle cells).
- The role of gene expression in determining cell fate.
- Cellular responses during embryonic development, tissue repair, and regeneration.
- Study of developmental disorders caused by defects in cellular differentiation.
Importance of These Sub-Areas of Cell Biology
These sub-areas form the foundation of cell biology and intersect with other disciplines such as genetics, molecular biology, and biophysics. They are essential for understanding normal cellular processes and identifying the mechanisms underlying diseases like cancer, genetic disorders, and autoimmune conditions. Advances in these sub-areas also drive innovations in biotechnology, medicine, and environmental science.
Below is an example of how the understanding of cellular processes is very important in developing cancer treatment:
A Case Study on HER2-Positive Breast Cancer
HER2 (Human Epidermal Growth Factor Receptor 2) is a protein that helps breast cancer cells grow quickly. Breast cancer cells with higher than normal levels of HER2 are called HER2-positive.
One of the most significant breakthroughs in cancer treatment has been understanding the role of the HER2/neu signaling pathway in HER2-positive breast cancer. This type of breast cancer is characterized by the overexpression of the HER2 protein, a receptor on the surface of cells that drives uncontrolled growth and division. This happens when the HER2 gene becomes amplified, meaning it produces too many copies of itself. These extra HER2 genes instruct breast cells to produce excessive HER2 receptors, leading to HER2 protein overexpression.
Thanks to decades of cellular and molecular research, targeted therapies have been developed to block HER2 activity. For instance, monoclonal antibodies like trastuzumab (Herceptin) bind to HER2 receptors, inhibiting their signaling and helping the immune system destroy cancer cells. This strategy is a direct application of insights from cell signaling and molecular biology. You can learn more about HER2-targeted treatments from resources such as the National Cancer Institute’s hormone therapy overview.
The Cellular Process: HER2 Signaling Pathway
- Normal Function: Under normal conditions, HER2 helps regulate cell growth by activating signaling pathways like PI3K/AKT and MAPK when a growth factor binds to the receptor.
- Cancerous Mutation: In HER2-positive breast cancer, the HER2 gene is amplified, resulting in an excessive number of HER2 receptors. This leads to overactive signaling even in the absence of growth factors, driving rapid and uncontrolled cell division—a hallmark of cancer.
The Treatment: Targeted Therapy with Trastuzumab (Herceptin)
Understanding the HER2 signaling pathway led to the development of trastuzumab, a monoclonal antibody that specifically targets the HER2 receptor. This form of targeted therapy has significantly improved outcomes for patients with HER2-positive breast cancer by interfering directly with the molecular mechanism driving tumor growth.
Mechanism of Action:
- HER2 Receptor Blocking: Trastuzumab binds to the HER2 receptors on the cancer cell surface, preventing receptor dimerization and subsequent activation of downstream signaling pathways that promote cell proliferation.
- Immune System Activation: The antibody-coated cancer cells become a target for the body’s immune system. Trastuzumab facilitates antibody-dependent cellular cytotoxicity (ADCC), where immune cells such as natural killer (NK) cells recognize and kill the tumor cells.
- Reduction of HER2 Expression: Treatment with trastuzumab has been shown to decrease the number of HER2 receptors on cancer cells, further weakening the aberrant signaling that drives tumor growth.
Impact on Treatment Outcomes for HER2-Positive Breast Cancer
- Improved Survival Rates: Patients with HER2-positive breast cancer treated with trastuzumab in combination with chemotherapy have significantly better survival rates compared to those treated with chemotherapy alone.
- Reduced Recurrence: Trastuzumab reduces the likelihood of cancer recurrence, particularly in early-stage HER2-positive breast cancer.
Broader Implications
The success of trastuzumab highlights how understanding a specific cellular process—HER2-mediated signaling—has transformed the treatment of a previously aggressive cancer subtype. It also paved the way for the development of other HER2-targeted therapies, such as pertuzumab and trastuzumab emtansine (T-DM1), further improving patient outcomes. For a deeper clinical overview, see the National Cancer Institute’s page on Herceptin.
Conclusion
The study of cellular processes, such as receptor-mediated signaling pathways, is pivotal in the fight against cancer. HER2-positive breast cancer serves as a powerful example of how targeting a specific cellular abnormality can lead to life-saving therapies, demonstrating the profound impact of cell biology on precision medicine.
Why Study Cell Biology
Understanding the Building Blocks of Life
Cell biology provides insight into the fundamental unit of life—the cell. By understanding how cells function, divide, and interact, students gain a clearer picture of the processes that sustain living organisms. This foundational knowledge supports all areas of biology and medicine.
Relevance to Human Health
Many diseases, including cancer and genetic disorders, originate from cellular dysfunctions. Studying cell biology enables students to understand the mechanisms behind these conditions. This is essential for those pursuing careers in healthcare, pharmaceuticals, or biomedical research.
Applications in Biotechnology
Cell biology is integral to advancements in biotechnology, such as gene editing, regenerative medicine, and vaccine development. Understanding cellular processes allows scientists to manipulate cells for beneficial purposes. This opens doors to innovation and entrepreneurship in the biotech industry.
Laboratory and Analytical Skills
Students of cell biology develop practical skills in microscopy, cell culturing, and molecular techniques. These skills are essential for research and diagnostics in various scientific fields. Hands-on experience enhances both academic learning and career readiness.
Preparation for Advanced Studies
A strong foundation in cell biology is crucial for further studies in molecular biology, genetics, immunology, and medicine. It provides the context and vocabulary for understanding more complex biological systems. This makes it a vital subject for any student pursuing life sciences.
Cell Biology: Review Questions with Detailed Answers
Q1. What are the main differences between prokaryotic and eukaryotic cells in terms of structure and complexity?
Answer:
Prokaryotic Cells:
- Nucleus: Lack a true nucleus; DNA resides in a nucleoid region.
- Organelles: Do not have membrane-bound organelles. Possess ribosomes (70S) for protein synthesis.
- Size: Generally smaller (1-10 micrometers in diameter).
- Reproduction: Reproduce asexually through binary fission.
- Examples: Bacteria and archaea.
- Cell Wall: Typically have a rigid cell wall composed of peptidoglycan (in bacteria).
Eukaryotic Cells:
- Nucleus: Have a true nucleus enclosed by a nuclear membrane; DNA is organized into chromosomes.
- Organelles: Contain membrane-bound organelles such as mitochondria, endoplasmic reticulum, Golgi apparatus, lysosomes, and, in plant cells, chloroplasts.
- Size: Generally larger (10-100 micrometers in diameter).
- Reproduction: Reproduce both sexually (meiosis) and asexually (mitosis).
- Examples: Animal cells, plant cells, fungi, and protists.
- Cell Wall: Plant cells have cell walls made of cellulose; animal cells lack a cell wall.
Impact on Functionality:
- Complexity: Eukaryotic cells can perform more complex functions due to compartmentalization provided by organelles.
- Genetic Regulation: Eukaryotes have more intricate mechanisms for gene regulation, allowing for cellular differentiation and multicellularity.
- Metabolic Processes: Specialized organelles enable diverse metabolic activities, such as ATP production in mitochondria and photosynthesis in chloroplasts.
Q2. Describe the structure and function of the mitochondria in eukaryotic cells.
Answer:
Mitochondria Structure:
- Double Membrane: Comprises an outer membrane and a highly folded inner membrane known as cristae.
- Matrix: The innermost compartment containing enzymes, mitochondrial DNA (mtDNA), ribosomes, and metabolites.
- Cristae: Increase the surface area for biochemical reactions, particularly those involved in energy production.
- Intermembrane Space: The region between the outer and inner membranes, involved in the electron transport chain.
Mitochondria Function:
Energy Production (ATP Synthesis):
- Cellular Respiration: Site of the citric acid cycle (Krebs cycle) and the electron transport chain, which convert biochemical energy from nutrients into ATP.
- Oxidative Phosphorylation: Utilizes the proton gradient created by the electron transport chain to drive ATP synthesis via ATP synthase.
Regulation of Metabolic Processes:
- Fatty Acid Oxidation: Breaks down fatty acids to generate acetyl-CoA for the citric acid cycle.
- Amino Acid Metabolism: Involved in deamination and other amino acid processing.
Apoptosis (Programmed Cell Death):
- Releases cytochrome c and other pro-apoptotic factors to trigger apoptosis, essential for development and cellular homeostasis.
Calcium Homeostasis:
- Regulates intracellular calcium levels, important for various signaling pathways.
Heat Production:
- In brown adipose tissue, mitochondria generate heat through non-shivering thermogenesis by uncoupling ATP synthesis from electron transport.
Significance:
- Energy Supply: Provides the majority of ATP required for cellular activities.
- Genetic Heritage: Mitochondria have their own DNA, supporting the endosymbiotic theory that mitochondria originated from free-living prokaryotes.
- Disease Association: Dysfunctional mitochondria are linked to various diseases, including metabolic disorders and neurodegenerative diseases.
Q3. How does the cell membrane regulate the movement of substances into and out of the cell?
Answer:
Cell Membrane Structure:
- Phospholipid Bilayer: Composed of hydrophilic heads facing outward and hydrophobic tails facing inward, providing a semi-permeable barrier.
- Proteins: Integral and peripheral proteins facilitate transport, signal transduction, and structural support.
- Carbohydrates: Attached to proteins and lipids, involved in cell recognition and communication.
- Cholesterol: Embedded within the bilayer, modulating fluidity and stability.
Regulation Mechanisms:
Passive Transport:
- Diffusion: Movement of molecules from high to low concentration without energy (e.g., oxygen and carbon dioxide).
- Facilitated Diffusion: Uses carrier proteins or channels to transport molecules like glucose and ions down their concentration gradient.
- Osmosis: Diffusion of water through aquaporin channels from areas of low solute concentration to high solute concentration.
Active Transport:
- Primary Active Transport: Utilizes ATP to move substances against their concentration gradient via pumps (e.g., Na⁺/K⁺-ATPase).
- Secondary Active Transport: Uses the electrochemical gradient created by primary active transport to drive the movement of other molecules (e.g., symport and antiport systems).
Bulk Transport:
- Endocytosis: Ingestion of large particles by forming vesicles (e.g., phagocytosis and pinocytosis).
- Exocytosis: Expulsion of materials from the cell by vesicle fusion with the plasma membrane.
Selective Permeability:
- Size and Polarity: Only small, nonpolar molecules can easily diffuse through the lipid bilayer, while larger or polar molecules require transport proteins.
- Charge: Ions and charged molecules cannot pass through the hydrophobic core without assistance.
Regulation by Membrane Proteins:
- Carrier Proteins: Bind specific molecules and undergo conformational changes to transport them across the membrane.
- Channel Proteins: Provide passageways for ions and water, often gated by stimuli like voltage or ligands.
- Receptor Proteins: Detect external signals and initiate cellular responses, influencing membrane permeability.
Importance of Regulation:
- Homeostasis: Maintains internal conditions by controlling nutrient uptake, waste removal, and ion balance.
- Cell Signaling: Regulates communication with other cells and responses to environmental changes.
- Energy Efficiency: Optimizes energy use by minimizing unnecessary transport and maintaining gradients essential for cellular functions.
Conclusion: The cell membrane’s complex structure and various transport mechanisms enable precise regulation of substance movement, essential for maintaining cellular integrity, functionality, and overall homeostasis.
Q4. Explain the phases of the cell cycle and the key events that occur in each phase.
Answer:
Cell Cycle Overview: The cell cycle is the series of events that take place in a cell leading to its division and duplication. It consists of interphase (G₁, S, G₂ phases) and the mitotic phase (mitosis and cytokinesis).
Phases of the Cell Cycle:
Interphase:
G₁ Phase (Gap 1):
- Duration: Variable; typically the longest phase.
- Events: Cell growth, protein synthesis, and organelle duplication. The cell performs normal metabolic functions and prepares for DNA replication.
- Checkpoints: G₁ checkpoint ensures the cell is ready for DNA synthesis and has adequate resources.
S Phase (Synthesis):
- Duration: Relatively short.
- Events: DNA replication occurs, resulting in the duplication of chromosomes. Each chromosome now consists of two sister chromatids.
- Significance: Ensures each daughter cell receives an identical set of chromosomes.
G₂ Phase (Gap 2):
- Duration: Shorter than G₁.
- Events: Further cell growth and protein synthesis. The cell prepares for mitosis by synthesizing proteins required for chromosome manipulation and division.
- Checkpoints: G₂ checkpoint verifies that DNA replication in S phase was successful and that the cell is ready to enter mitosis.
Mitotic Phase (M Phase):
- Mitosis:
- Purpose: Division of the cell nucleus, ensuring equal distribution of chromosomes to daughter cells.
- Subphases:
- Prophase:
- Chromatin condenses into visible chromosomes.
- The nuclear envelope begins to disintegrate.
- Mitotic spindle fibers start to form from the centrosomes.
- Metaphase:
- Chromosomes align at the cell’s equatorial plane (metaphase plate).
- Spindle fibers attach to the centromeres of chromosomes via kinetochores.
- Anaphase:
- Sister chromatids separate at the centromeres.
- Spindle fibers shorten, pulling the chromatids toward opposite poles of the cell.
- Telophase:
- Chromatids arrive at the poles and decondense back into chromatin.
- Nuclear envelopes re-form around each set of chromosomes, creating two distinct nuclei.
- Prophase:
- Cytokinesis:
- Purpose: Division of the cell’s cytoplasm, resulting in two separate daughter cells.
- Process:
- Animal Cells: A cleavage furrow forms, pinching the cell membrane inward until the cell splits.
- Plant Cells: A cell plate forms along the equator, developing into a new cell wall that separates the two daughter cells.
- Mitosis:
Checkpoints and Regulation:
- G₁ Checkpoint: Determines if the cell has enough resources and is appropriate to enter S phase.
- G₂ Checkpoint: Ensures DNA replication is complete and accurate before entering mitosis.
- M Checkpoint (Spindle Assembly Checkpoint): Confirms that all chromosomes are properly attached to the spindle fibers and aligned before proceeding with anaphase.
Significance of the Cell Cycle:
- Growth and Development: Essential for the growth of multicellular organisms and the replacement of worn-out cells.
- Genetic Stability: Proper regulation and accurate DNA replication prevent mutations and maintain genetic integrity across generations of cells.
- Cancer Connection: Dysregulation of the cell cycle checkpoints can lead to uncontrolled cell division, resulting in cancer.
Conclusion: The cell cycle is a meticulously regulated process that ensures cells grow, replicate their DNA accurately, and divide to produce genetically identical daughter cells. Understanding each phase and its key events is fundamental to comprehending cellular biology, growth, and the development of diseases like cancer.
Q5. What are ribosomes, and how do they facilitate protein synthesis in the cell?
Answer:
Ribosomes Overview: Ribosomes are complex molecular machines composed of ribosomal RNA (rRNA) and proteins. They are the sites of protein synthesis (translation) in both prokaryotic and eukaryotic cells.
Structure of Ribosomes:
- Subunits:
- Prokaryotes: Composed of a small 30S subunit and a large 50S subunit, forming a 70S ribosome.
- Eukaryotes: Composed of a small 40S subunit and a large 60S subunit, forming an 80S ribosome.
- Composition: Ribosomes consist of rRNA molecules and various ribosomal proteins. The rRNA plays a crucial role in the catalytic activity of the ribosome.
Function in Protein Synthesis:
mRNA Binding:
- The small ribosomal subunit binds to the messenger RNA (mRNA) at the start codon (AUG).
- The large subunit then joins, forming a complete ribosome with designated A (aminoacyl), P (peptidyl), and E (exit) sites.
tRNA Interaction:
- Transfer RNA (tRNA) molecules carry specific amino acids to the ribosome.
- Each tRNA has an anticodon that is complementary to the codon on the mRNA, ensuring the correct amino acid is added to the growing polypeptide chain.
Peptide Bond Formation:
- The ribosome catalyzes the formation of peptide bonds between adjacent amino acids, facilitated by the ribosomal RNA’s catalytic sites.
- The growing polypeptide chain is transferred from the tRNA in the P site to the amino acid on the tRNA in the A site.
Translocation:
- The ribosome moves along the mRNA, shifting the peptidyl-tRNA from the A site to the P site and freeing the A site for the next aminoacyl-tRNA.
- This process continues, adding amino acids sequentially to the polypeptide chain.
Termination:
- When a stop codon (UAA, UAG, UGA) is encountered, release factors bind to the ribosome.
- The completed polypeptide chain is released, and the ribosomal subunits dissociate from the mRNA and each other.
Types of Ribosomes:
- Free Ribosomes: Located in the cytoplasm, synthesizing proteins that function within the cell.
- Bound Ribosomes: Attached to the endoplasmic reticulum (ER), specifically the rough ER, synthesizing proteins destined for secretion, membrane insertion, or lysosomal storage.
Regulation of Ribosome Function:
- Ribosome Biogenesis: Ribosomes are assembled in the nucleolus (in eukaryotes) where rRNA is transcribed and combined with ribosomal proteins.
- Quality Control: Cells monitor ribosome assembly to ensure functionality and prevent the accumulation of defective ribosomes.
- Regulatory Factors: Initiation factors, elongation factors, and release factors regulate different stages of translation, ensuring accuracy and efficiency.
Significance of Ribosomes:
- Protein Diversity: Ribosomes enable the synthesis of a vast array of proteins, essential for virtually all cellular functions.
- Genetic Expression: They translate the genetic information encoded in mRNA into functional proteins, linking genotype to phenotype.
- Antibiotic Targets: Many antibiotics (e.g., tetracyclines, macrolides) target bacterial ribosomes, exploiting differences between prokaryotic and eukaryotic ribosomes to inhibit bacterial protein synthesis without affecting human cells.
Conclusion: Ribosomes are indispensable for translating genetic information into functional proteins, acting as the machinery that synthesizes the proteins necessary for cell structure, function, and regulation. Their precise interaction with mRNA and tRNA ensures accurate protein synthesis, essential for the maintenance and operation of all living cells.
Q6. What is the role of the endoplasmic reticulum in cellular transport and protein modification?
Answer:
Endoplasmic Reticulum (ER) Overview: The endoplasmic reticulum is a network of membranous tubules and flattened sacs within the cytoplasm of eukaryotic cells. It plays a critical role in the synthesis, folding, modification, and transport of proteins and lipids.
Types of Endoplasmic Reticulum:
Rough Endoplasmic Reticulum (RER):
- Structure: Studded with ribosomes on its cytoplasmic surface, giving it a “rough” appearance.
- Function:
- Protein Synthesis: Ribosomes on the RER synthesize proteins destined for secretion, incorporation into membranes, or lysosomes.
- Protein Folding and Quality Control: Chaperone proteins within the RER assist in proper protein folding and ensure quality control by targeting misfolded proteins for degradation.
- Post-Translational Modifications: Proteins undergo modifications such as glycosylation (addition of carbohydrate groups) and disulfide bond formation, which are essential for their stability and function.
Smooth Endoplasmic Reticulum (SER):
- Structure: Lacks ribosomes, giving it a “smooth” appearance.
- Function:
- Lipid Synthesis: Synthesizes lipids, including phospholipids and cholesterol, which are essential components of cellular membranes.
- Detoxification: Metabolizes and detoxifies drugs, toxins, and metabolic waste products, particularly in liver cells.
- Calcium Storage: Stores and regulates intracellular calcium ions, important for various signaling pathways and muscle contraction.
- Carbohydrate Metabolism: Involved in processes like gluconeogenesis (production of glucose from non-carbohydrate sources).
Cellular Transport:
- Vesicle Formation: The ER forms vesicles that transport synthesized proteins and lipids to other parts of the cell.
- Golgi Apparatus Interaction: Vesicles bud off from the ER and fuse with the Golgi apparatus for further processing, sorting, and packaging before delivery to their final destinations.
- Membrane Protein Integration: Proteins destined for the plasma membrane or organelle membranes are inserted into the ER membrane during synthesis and subsequently trafficked to their target locations.
Protein Modification:
- Glycosylation: Addition of carbohydrate moieties to proteins, which is crucial for protein folding, stability, and cell-cell recognition.
- Disulfide Bond Formation: Formation of covalent bonds between cysteine residues, stabilizing the three-dimensional structure of proteins.
- Proteolytic Cleavage: Removal of signal peptides or propeptides from nascent proteins to activate them or target them to specific cellular locations.
- Quality Control Mechanisms: ER-associated degradation (ERAD) pathways identify and degrade misfolded or improperly assembled proteins, maintaining proteostasis within the cell.
Significance in the Cell:
- Protein Quality Assurance: Ensures that only properly folded and functional proteins proceed through the secretory pathway, preventing cellular dysfunction caused by aberrant proteins.
- Membrane Composition: Contributes to the synthesis and maintenance of cellular membranes, essential for compartmentalization and organelle function.
- Detoxification Processes: Protects the cell from harmful substances by metabolizing and neutralizing toxins.
- Calcium Homeostasis: Regulates calcium levels, which are critical for various cellular processes, including signaling, muscle contraction, and enzyme activity.
Conclusion: The endoplasmic reticulum is vital for the synthesis, modification, and transport of proteins and lipids within the cell. Its specialized functions in protein folding, quality control, lipid metabolism, and detoxification are essential for maintaining cellular integrity, functionality, and homeostasis.
Q7. How do lysosomes contribute to cellular homeostasis, and what are the consequences of lysosomal dysfunction?
Answer:
Lysosomes Overview: Lysosomes are membrane-bound organelles found in eukaryotic cells, containing a variety of hydrolytic enzymes capable of breaking down all types of biological polymers—proteins, nucleic acids, carbohydrates, and lipids.
Functions of Lysosomes:
Intracellular Digestion:
- Autophagy: Degradation and recycling of the cell’s own damaged or obsolete organelles and macromolecules. Autophagosomes engulf the targeted components and fuse with lysosomes for breakdown.
- Endocytosis: Digestion of extracellular materials brought into the cell via endosomes. Phagocytosis (engulfing large particles) and pinocytosis (ingesting fluids) lead to the formation of phagosomes and pinocytic vesicles, respectively, which then fuse with lysosomes.
Waste Removal:
- Macromolecule Breakdown: Lysosomal enzymes break down macromolecules into their monomeric units, which are then transported out of the lysosome and reused by the cell.
Defense Mechanism:
- Pathogen Destruction: In immune cells like macrophages and neutrophils, lysosomes digest engulfed pathogens such as bacteria and viruses, contributing to the body’s defense against infections.
Cell Signaling and Apoptosis:
- Signaling Pathways: Lysosomes participate in cellular signaling processes, including nutrient sensing and energy homeostasis.
- Programmed Cell Death: Lysosomal enzymes can be released into the cytoplasm during apoptosis, aiding in the controlled dismantling of the cell.
Lysosomal Enzymes:
- Hydrolytic Enzymes: Include proteases, lipases, nucleases, and carbohydrases that function optimally at acidic pH levels (around 4.5-5.0) within the lysosome.
Consequences of Lysosomal Dysfunction:
Lysosomal Storage Diseases:
- Definition: Genetic disorders caused by deficiencies in specific lysosomal enzymes, leading to the accumulation of undigested substrates.
- Examples:
- Gaucher Disease: Deficiency in glucocerebrosidase leads to the accumulation of glucocerebroside in macrophages.
- Tay-Sachs Disease: Deficiency in hexosaminidase A results in the buildup of GM2 gangliosides in neurons.
- Pompe Disease: Deficiency in acid alpha-glucosidase causes glycogen accumulation in muscle cells.
Cellular Damage and Death:
- Accumulation of Waste: Undigested substrates can disrupt cellular function, leading to organelle malfunction and impaired metabolism.
- Autophagic Blockage: Incomplete autophagy can result in the accumulation of damaged organelles, contributing to cellular stress and apoptosis.
- Apoptosis Induction: Excessive release of lysosomal enzymes into the cytoplasm can trigger uncontrolled cell death.
Neurodegenerative Diseases:
- Alzheimer’s Disease: Impaired lysosomal function is associated with the accumulation of amyloid-beta plaques and tau tangles.
- Parkinson’s Disease: Dysfunctional lysosomes contribute to the buildup of alpha-synuclein aggregates.
Immune System Impairment:
- Reduced Pathogen Clearance: Defective lysosomes in immune cells hinder the digestion of engulfed pathogens, compromising the body’s ability to fight infections.
Mechanisms to Prevent Lysosomal Dysfunction:
- Proper Enzyme Targeting: Lysosomal enzymes are tagged with mannose-6-phosphate in the Golgi apparatus to ensure correct delivery to lysosomes.
- Quality Control: Cells employ quality control mechanisms to detect and degrade misfolded or defective lysosomal enzymes.
- Autophagy Regulation: Ensures efficient removal of damaged organelles and proteins, preventing toxic accumulations.
Conclusion: Lysosomes are essential for maintaining cellular homeostasis through the degradation and recycling of macromolecules and damaged organelles. Dysfunction of lysosomes can lead to severe cellular and systemic consequences, including lysosomal storage diseases, neurodegeneration, and compromised immune function. Understanding lysosomal biology is crucial for diagnosing and developing treatments for these disorders.
Q8. What is the significance of the cytoskeleton in maintaining cell shape and facilitating cellular movement?
Answer:
Cytoskeleton Overview: The cytoskeleton is a dynamic network of protein fibers within the cytoplasm of eukaryotic cells. It provides structural support, maintains cell shape, facilitates cellular movement, and plays roles in intracellular transport and cell division.
Components of the Cytoskeleton:
Microfilaments (Actin Filaments):
- Structure: Thin (7 nm) helical fibers composed of actin monomers.
- Function:
- Cell Shape Maintenance: Provide mechanical support and determine cell morphology.
- Cell Movement: Involved in amoeboid movement, muscle contraction, and the formation of cellular extensions like lamellipodia and filopodia.
- Intracellular Transport: Facilitate the movement of organelles and vesicles within the cell through motor proteins like myosin.
Intermediate Filaments:
- Structure: Medium (10 nm) sturdy fibers made from various proteins (e.g., keratins, vimentin).
- Function:
- Mechanical Stability: Resist mechanical stress and maintain the integrity of the cell, especially in tissues subjected to tension (e.g., epithelial cells).
- Nuclear Support: Anchor the nucleus and organize the nuclear architecture.
Microtubules:
- Structure: Thick (25 nm) hollow tubes composed of tubulin dimers (α and β tubulin).
- Function:
- Cell Shape and Structure: Provide rigidity and support to the cell.
- Intracellular Transport: Serve as tracks for motor proteins like kinesin and dynein to transport vesicles, organelles, and other cargo.
- Cell Division: Form the mitotic spindle, which segregates chromosomes during mitosis and meiosis.
- Cilia and Flagella Formation: Constitute the core structure of cilia and flagella, enabling cell movement and fluid movement across cell surfaces.
Significance in Maintaining Cell Shape:
- Structural Support: The cytoskeleton provides a framework that resists deformation and maintains the cell’s shape against external forces.
- Flexibility and Rigidity: While microfilaments and microtubules offer rigidity, the dynamic nature of the cytoskeleton allows cells to change shape when necessary, such as during migration or division.
- Compartmentalization: Helps organize and anchor organelles within the cell, maintaining spatial relationships and facilitating efficient cellular function.
Facilitation of Cellular Movement:
- Amoeboid Movement: Actin polymerization at the leading edge of the cell drives protrusion of pseudopodia, allowing the cell to crawl.
- Muscle Contraction: Actin and myosin filaments slide past each other to generate muscle contractions.
- Intracellular Transport: Microtubules provide tracks for vesicles and organelles to move to their designated locations within the cell.
- Cilia and Flagella: Microtubule-based structures generate wave-like movements that propel cells or move substances along cell surfaces (e.g., cilia in respiratory epithelium).
Dynamic Nature of the Cytoskeleton:
- Polymerization and Depolymerization: The cytoskeleton can rapidly assemble and disassemble its components in response to cellular signals, allowing adaptability and responsiveness.
- Regulatory Proteins: Various proteins regulate the stability, growth, and interaction of cytoskeletal fibers, ensuring precise control over cellular processes.
Consequences of Cytoskeletal Dysfunction:
- Cell Shape Abnormalities: Impaired cytoskeleton can lead to distorted cell shapes, affecting tissue structure and function.
- Movement Impairment: Defects in cytoskeletal components can hinder cell migration, impacting processes like wound healing and immune responses.
- Transport Defects: Disruptions in microtubule tracks can impede the movement of organelles and vesicles, affecting intracellular logistics.
- Cell Division Errors: Malfunctioning mitotic spindles can lead to improper chromosome segregation, resulting in aneuploidy and genomic instability.
Conclusion: The cytoskeleton is indispensable for maintaining cell shape, providing mechanical support, and enabling a wide range of cellular movements. Its dynamic and regulated nature allows cells to adapt to changing environments and perform essential functions. Understanding the cytoskeleton’s structure and roles is fundamental to comprehending cellular mechanics, development, and the basis of various diseases related to cytoskeletal defects.
Q9. How do the processes of endocytosis and exocytosis facilitate communication and material exchange between the cell and its environment?
Answer:
Endocytosis and Exocytosis Overview: Endocytosis and exocytosis are active transport processes that regulate the movement of large molecules, particles, and even whole cells across the plasma membrane, enabling dynamic interactions between the cell and its environment.
Endocytosis:
- Definition: The process by which cells internalize extracellular materials by engulfing them with the plasma membrane, forming vesicles.
- Types of Endocytosis:
- Phagocytosis (“Cell Eating”):
- Process: Engulfment of large particles such as bacteria, dead cells, or debris.
- Example: Macrophages and neutrophils ingest pathogens and cellular debris as part of the immune response.
- Pinocytosis (“Cell Drinking”):
- Process: Uptake of extracellular fluid and dissolved solutes through small vesicles.
- Example: Cells continuously absorb nutrients and fluids from their surroundings via micropinocytosis.
- Receptor-Mediated Endocytosis:
- Process: Specific molecules bind to receptors on the plasma membrane, triggering vesicle formation.
- Example: Uptake of cholesterol via LDL receptors or internalization of growth factors like EGF (epidermal growth factor).
- Phagocytosis (“Cell Eating”):
- Mechanism:
- Vesicle Formation: The plasma membrane invaginates to encase the target material, pinching off to form an internal vesicle.
- Vesicle Trafficking: Internalized vesicles are transported to specific intracellular destinations, such as lysosomes for digestion or the Golgi apparatus for processing.
Exocytosis:
Definition: The process by which cells expel materials to the extracellular environment by fusing vesicles with the plasma membrane.
Types of Exocytosis:
- Constitutive Exocytosis:
- Process: Continuous delivery and secretion of membrane proteins, lipids, and extracellular matrix components.
- Example: Release of digestive enzymes from pancreatic cells into the digestive tract.
- Regulated Exocytosis:
- Process: Controlled release of specific substances in response to stimuli.
- Example: Secretion of neurotransmitters from neurons in response to an action potential or release of insulin from pancreatic beta cells in response to high blood glucose levels.
- Constitutive Exocytosis:
Mechanism:
- Vesicle Trafficking: Vesicles containing the material to be secreted move to the plasma membrane.
- Membrane Fusion: Vesicles dock and fuse with the plasma membrane, releasing their contents outside the cell.
- Incorporation of Membrane Components: The vesicle membrane merges with the plasma membrane, integrating proteins and lipids into the cell’s outer layer.
Facilitation of Communication and Material Exchange:
Signal Transduction:
- Receptor Recycling: Receptor-mediated endocytosis allows cells to regulate the number of receptors on the surface, modulating sensitivity to signaling molecules.
- Downstream Signaling: Internalized receptors can continue to signal from endosomes, influencing cellular responses.
Nutrient Uptake:
- Essential Molecules: Endocytosis enables cells to acquire large nutrients, such as lipoproteins (e.g., LDL) and complex carbohydrates, which cannot passively diffuse through the membrane.
Waste Removal:
- Expulsion of Waste: Exocytosis facilitates the removal of metabolic waste products and harmful substances from the cell, maintaining intracellular homeostasis.
Secretion of Proteins and Hormones:
- Cellular Communication: Exocytosis allows the release of hormones, neurotransmitters, and other signaling molecules that mediate communication between cells and tissues.
Membrane Expansion and Repair:
- Membrane Recycling: Exocytosis contributes to membrane growth during cell division and the repair of membrane damage by replenishing lost membrane components.
Pathogen Entry and Immune Response:
- Phagocytosis: Engulfing pathogens helps in their destruction and presentation to the immune system, initiating immune responses.
- Exocytosis of Antibodies: Immune cells use exocytosis to release antibodies and antimicrobial agents to neutralize pathogens.
Consequences of Dysregulation:
- Impaired Nutrient Uptake: Defects in endocytosis can lead to deficiencies in essential nutrients and receptors, affecting cell function and signaling.
- Excessive Secretion: Overactive exocytosis may result in the excessive release of hormones or neurotransmitters, disrupting physiological balance.
- Immune Dysfunction: Inefficient phagocytosis can compromise the immune system’s ability to eliminate pathogens.
- Cellular Stress: Accumulation of waste products or failure to remove toxins can lead to cellular damage and apoptosis.
Conclusion: Endocytosis and exocytosis are pivotal processes that enable cells to interact with their environment, facilitating the uptake of essential materials, removal of waste, and communication with other cells. Proper regulation of these mechanisms is essential for maintaining cellular homeostasis, effective communication, and overall organismal health.
Q10. What are the different types of cell junctions, and how do they contribute to tissue structure and function?
Answer:
Cell Junctions Overview: Cell junctions are specialized structures that connect adjacent cells in tissues, providing mechanical stability, facilitating communication, and regulating the movement of substances between cells. They are essential for maintaining the integrity and functionality of multicellular organisms.
Types of Cell Junctions:
Tight Junctions (Occluding Junctions):
- Structure: Composed of claudins and occludins that form a seal between adjacent cells near their apical surfaces.
- Function:
- Barrier Formation: Prevents the free passage of molecules and ions through the space between cells, maintaining distinct extracellular environments.
- Apical-Basal Polarity: Helps establish and maintain the polarity of epithelial cells by preventing the mixing of apical and basolateral membrane proteins.
- Location: Predominantly found in epithelial tissues lining organs and body cavities (e.g., intestinal epithelium, blood-brain barrier).
Adherens Junctions (Zonula Adherens):
- Structure: Comprised of cadherin proteins linked to the actin cytoskeleton via catenins.
- Function:
- Mechanical Attachment: Provides strong mechanical bonds between cells, maintaining tissue integrity.
- Signal Transduction: Involved in transmitting signals that regulate cell behavior and differentiation.
- Location: Common in epithelial and endothelial tissues, forming a belt-like structure encircling the cell.
Desmosomes (Macula Adherens):
- Structure: Consist of cadherins (desmogleins and desmocollins) linked to intermediate filaments (keratin) via adaptor proteins (desmoplakins and plakoglobins).
- Function:
- Mechanical Strength: Distribute tensile forces across tissues, preventing cells from tearing apart under stress.
- Tissue Integrity: Essential for maintaining the structural integrity of tissues subjected to mechanical stress, such as skin and cardiac muscle.
- Location: Abundant in tissues like the epidermis, heart muscle, and uterus.
Gap Junctions:
- Structure: Composed of connexin proteins that form channels (connexons) allowing direct cytoplasmic communication between adjacent cells.
- Function:
- Electrical and Chemical Coupling: Permit the passage of ions, metabolites, and signaling molecules, enabling coordinated cellular activities.
- Synchronization: Essential for synchronized muscle contractions and coordinated responses in neuronal networks.
- Location: Found in cardiac muscle, smooth muscle, neurons, and various epithelial tissues.
Hemidesmosomes:
- Structure: Similar to desmosomes but connect cells to the extracellular matrix instead of adjacent cells. Composed of integrins linked to intermediate filaments.
- Function:
- Anchoring: Secure epithelial cells to the underlying basement membrane, providing stability and resistance to shear stress.
- Location: Prominently present in epithelial tissues, such as the skin’s basal layer.
Anchoring Junctions:
- Includes: Desmosomes and hemidesmosomes.
- Function: Provide strong adhesion between cells and between cells and the extracellular matrix, ensuring tissue resilience.
Contribution to Tissue Structure and Function:
Mechanical Stability:
- Tissue Integrity: Desmosomes and adherens junctions distribute mechanical stress, preventing tissue damage during movement and contraction.
- Barrier Function: Tight junctions maintain distinct environments on either side of epithelial layers, essential for organ function (e.g., absorption in the intestines).
Cell Communication:
- Signal Transduction: Adherens junctions participate in transmitting signals that influence cell growth, differentiation, and apoptosis.
- Coordinated Activity: Gap junctions enable cells to function as a synchronized unit, crucial for heart contractions and neuronal signaling.
Cell Polarization:
- Apical-Basal Orientation: Tight junctions establish and maintain cell polarity, which is vital for the directional transport of substances across epithelial layers.
Tissue Differentiation and Development:
- Morphogenesis: Cell junctions play roles in shaping tissues during development by regulating cell adhesion and movement.
- Stem Cell Niche Maintenance: Provide the structural and signaling environment necessary for stem cell maintenance and differentiation.
Immune Response:
- Barrier Protection: Tight junctions and desmosomes in epithelial barriers prevent pathogen entry, contributing to the body’s defense mechanisms.
Consequences of Junction Dysfunction:
- Loss of Tissue Integrity: Impaired cell junctions can lead to weakened tissues, making them susceptible to damage and disease.
- Barrier Dysfunction: Compromised tight junctions can result in leaky barriers, allowing pathogens and toxins to enter tissues (e.g., leaky gut syndrome).
- Cardiac Arrhythmias: Dysfunctional gap junctions in the heart can disrupt electrical signaling, leading to arrhythmias.
- Skin Disorders: Defects in desmosomes can cause skin blistering diseases, such as pemphigus vulgaris.
Conclusion: Cell junctions are essential for maintaining the structural integrity, communication, and functionality of tissues. Each type of junction plays specific roles in connecting cells to each other and to the extracellular matrix, ensuring coordinated and resilient multicellular organisms. Understanding cell junctions is crucial for comprehending tissue organization, development, and the pathology of various diseases.
Q11. How do mitochondria and chloroplasts exemplify the endosymbiotic theory, and what evidence supports this theory?
Answer:
Endosymbiotic Theory Overview: The endosymbiotic theory proposes that mitochondria and chloroplasts originated as free-living prokaryotes that were engulfed by ancestral eukaryotic cells. This symbiotic relationship became permanent, leading to the evolution of modern eukaryotic cells.
Key Features Exemplifying Endosymbiotic Theory:
Double Membrane Structure:
- Mitochondria and Chloroplasts: Both organelles possess a double membrane, consistent with the engulfing mechanism where the outer membrane originates from the host cell and the inner membrane from the engulfed prokaryote.
Own Genetic Material:
- Mitochondria: Contain their own circular DNA (mtDNA), resembling bacterial genomes.
- Chloroplasts: Possess their own circular DNA, similar to that of cyanobacteria.
- Evidence: The genetic sequences of mitochondrial and chloroplast DNA are more closely related to certain prokaryotes than to the nuclear DNA of the host eukaryotic cell.
Ribosomes:
- Similarity to Bacterial Ribosomes: Mitochondria and chloroplasts have ribosomes (70S) that resemble those of prokaryotes, distinct from the 80S ribosomes found in the eukaryotic cytoplasm.
- Protein Synthesis: These organelles can synthesize some of their own proteins independently of the host cell’s ribosomes.
Reproduction Mechanism:
- Binary Fission: Mitochondria and chloroplasts replicate independently within the cell through a process similar to bacterial binary fission, maintaining their numbers in daughter cells during cell division.
Phylogenetic Evidence:
- Genetic Sequencing: Comparative analyses of DNA and protein sequences show that mitochondria are closely related to alpha-proteobacteria, while chloroplasts are related to cyanobacteria.
- Evolutionary Trees: Phylogenetic trees place mitochondria and chloroplasts within the bacterial domains, supporting their prokaryotic origins.
Functional Complementarity:
- Energy Conversion: Mitochondria and chloroplasts perform essential energy-converting processes (cellular respiration and photosynthesis, respectively) that benefit the host cell, indicating a mutually beneficial relationship.
Presence in Eukaryotes:
- Universal Distribution: All eukaryotic organisms possess mitochondria, and photosynthetic eukaryotes also contain chloroplasts, suggesting a common endosymbiotic origin.
Supporting Evidence:
Morphological Evidence:
- Size and Shape: Mitochondria and chloroplasts are similar in size and shape to certain bacteria.
- Internal Structures: Chloroplasts contain thylakoid membranes organized into stacks called grana, akin to the internal membranes of cyanobacteria.
Genetic Evidence:
- Gene Similarity: Mitochondrial and chloroplast genes share significant homology with bacterial genes, including genes involved in replication, transcription, and translation.
- Limited Gene Transfer: While some genes have been transferred to the host nucleus over evolutionary time, remnants of their original genomes persist within the organelles.
Biochemical Evidence:
- Electron Transport Chains: Mitochondrial and chloroplast electron transport chains have similarities to those found in bacteria.
- Fatty Acid Composition: The lipids in mitochondrial and chloroplast membranes resemble bacterial lipids, differing from those of the eukaryotic host.
Antibiotic Sensitivity:
- Sensitivity to Bacterial Antibiotics: Mitochondria and chloroplasts are sensitive to antibiotics that target bacterial ribosomes, supporting their prokaryotic characteristics.
Lateral Gene Transfer:
- Shared Pathways: Certain metabolic pathways and enzymes are shared between these organelles and bacteria, indicating a common evolutionary origin.
Implications of Endosymbiotic Theory:
- Evolution of Eukaryotes: The acquisition of mitochondria and chloroplasts was a pivotal event in the evolution of complex multicellular life, enabling greater energy efficiency and the development of diverse metabolic capabilities.
- Symbiotic Relationships: Highlights the importance of symbiosis in evolutionary history, demonstrating how cooperation between different organisms can drive major evolutionary transitions.
Conclusion: Mitochondria and chloroplasts exhibit numerous structural, genetic, and functional characteristics that strongly support the endosymbiotic theory. This theory provides a robust framework for understanding the origin of these essential organelles and the evolution of eukaryotic cells, illustrating the profound impact of symbiotic relationships in the history of life on Earth.
Q12. How does the cell cycle ensure genetic stability, and what mechanisms prevent the propagation of damaged DNA during cell division?
Answer:
Cell Cycle and Genetic Stability Overview: The cell cycle is a tightly regulated series of events that ensures cells grow, replicate their DNA accurately, and divide to produce genetically identical daughter cells. Maintaining genetic stability is crucial to prevent mutations, which can lead to diseases like cancer.
Mechanisms Ensuring Genetic Stability:
DNA Replication Accuracy:
- High-Fidelity DNA Polymerases: Enzymes responsible for DNA synthesis possess proofreading abilities, allowing them to detect and correct mismatched bases during replication.
- Error Rate Control: The intrinsic accuracy of DNA polymerases minimizes replication errors, maintaining genetic integrity.
Cell Cycle Checkpoints:
- G₁ Checkpoint:
- Function: Assesses cell size, nutrient availability, and DNA integrity before allowing entry into the S phase (DNA synthesis).
- Mechanism: If DNA damage is detected, the checkpoint halts the cell cycle to allow for repair or triggers apoptosis if damage is irreparable.
- G₂ Checkpoint:
- Function: Verifies that DNA replication in the S phase has been completed successfully and checks for any DNA damage before entering mitosis.
- Mechanism: Ensures that only cells with intact and fully replicated DNA proceed to division.
- M Checkpoint (Spindle Assembly Checkpoint):
- Function: Ensures that all chromosomes are properly attached to the mitotic spindle before anaphase proceeds.
- Mechanism: Prevents unequal chromosome segregation, thereby avoiding aneuploidy.
- G₁ Checkpoint:
DNA Repair Mechanisms:
- Mismatch Repair: Corrects base-pairing errors that escape proofreading during DNA replication.
- Base Excision Repair: Fixes small, non-helix-distorting base lesions.
- Nucleotide Excision Repair: Removes bulky helix-distorting lesions, such as thymine dimers caused by UV light.
- Double-Strand Break Repair:
- Homologous Recombination: Uses a sister chromatid as a template for accurate repair.
- Non-Homologous End Joining: Ligates broken DNA ends directly, which can be error-prone but essential for cell survival when homologous templates are unavailable.
Apoptosis (Programmed Cell Death):
- Function: Eliminates cells with excessive DNA damage or irreparable errors, preventing the propagation of mutations.
- Mechanism: Triggered by signals from damaged DNA or malfunctioning cell cycle regulators, leading to cell death without inflammation.
Tumor Suppressor Proteins:
- p53: Acts as a “guardian of the genome,” initiating cell cycle arrest, DNA repair, or apoptosis in response to DNA damage.
- Rb (Retinoblastoma Protein): Regulates the G₁ to S phase transition, preventing cells with damaged DNA from replicating.
Cyclins and Cyclin-Dependent Kinases (CDKs):
- Function: Regulate the progression of the cell cycle through various checkpoints by phosphorylating target proteins.
- Control: Proper timing and levels of cyclins ensure that cell cycle transitions occur only when conditions are favorable and DNA is intact.
Genomic Surveillance Systems:
- DNA Damage Response (DDR): A network of signaling pathways that detect DNA damage, signal its presence, and mediate repair processes.
- Checkpoint Kinases (e.g., Chk1 and Chk2): Transduce signals from damaged DNA to enforce cell cycle arrest and facilitate repair.
Prevention of Damaged DNA Propagation:
Halt Cell Cycle Progression:
- Temporary Arrest: Checkpoints can pause the cell cycle, providing time for DNA repair mechanisms to correct errors before replication or division.
Enhance DNA Repair:
- Recruitment of Repair Machinery: Checkpoints activate and localize DNA repair enzymes to the sites of damage.
Induce Apoptosis:
- Eliminate Faulty Cells: If DNA damage is beyond repair, checkpoints trigger apoptosis to remove the compromised cell from the population.
Prevent Aneuploidy:
- Accurate Chromosome Segregation: The spindle assembly checkpoint ensures that chromosomes are evenly and correctly distributed to daughter cells, preventing chromosomal abnormalities.
Consequences of Cell Cycle Dysregulation:
- Uncontrolled Cell Division: Loss of checkpoint control can lead to unchecked cell proliferation, a hallmark of cancer.
- Genetic Instability: Accumulation of DNA mutations increases the risk of malignant transformations and other genetic disorders.
- Developmental Defects: Errors in cell cycle regulation during development can result in congenital abnormalities and impaired tissue function.
Conclusion: The cell cycle incorporates multiple layers of regulation and repair mechanisms to ensure genetic stability. By employing checkpoints, DNA repair systems, and apoptotic pathways, cells can prevent the propagation of damaged DNA, maintaining the integrity of the genome and safeguarding the health of the organism.
These review questions and detailed answers cover essential topics in cell biology, including cell structure, organelle functions, cellular transport mechanisms, cell cycle regulation, and genetic stability. They are designed to enhance understanding, promote critical thinking, and reinforce key concepts for students studying cell biology and related disciplines
Thought-Provoking Questions on Cell Biology
1. What are the key structural differences between prokaryotic and eukaryotic cells, and how do these differences influence their respective functions?
Answer:
Prokaryotic Cells:
- Nucleus: Lack a true nucleus; instead, their genetic material resides in a region called the nucleoid.
- Organelles: Do not possess membrane-bound organelles. Ribosomes are present but smaller (70S) compared to eukaryotes.
- Size: Generally smaller (1-10 micrometers in diameter), allowing for rapid growth and reproduction.
- Cell Wall: Typically have a rigid cell wall composed of peptidoglycan (in bacteria) which provides structural support and protection.
- Reproduction: Primarily reproduce asexually through binary fission, enabling quick population growth.
- Genetic Material: Circular DNA molecule, often with additional small circular DNA called plasmids that can carry antibiotic resistance genes.
- Flagella: Structurally simpler, composed of the protein flagellin, allowing for basic motility.
Eukaryotic Cells:
- Nucleus: Possess a true nucleus enclosed by a double membrane (nuclear envelope), housing linear chromosomes.
- Organelles: Contain a variety of membrane-bound organelles such as mitochondria, endoplasmic reticulum (both rough and smooth), Golgi apparatus, lysosomes, and, in plant cells, chloroplasts.
- Size: Larger (10-100 micrometers in diameter), providing compartmentalization that allows for specialized functions.
- Cell Wall: In plants and fungi, cell walls are present (cellulose in plants, chitin in fungi), providing structural support. Animal cells lack a cell wall.
- Reproduction: Can reproduce both sexually (through meiosis and fertilization) and asexually (through mitosis), allowing for genetic diversity and complex life cycles.
- Genetic Material: Linear DNA organized into chromosomes, associated with histone proteins for packaging and regulation.
- Flagella and Cilia: More complex structures composed of microtubules arranged in a “9+2” pattern, enabling coordinated and efficient movement.
Influence on Functions:
- Compartmentalization: Eukaryotic cells can perform more complex and specialized functions due to the presence of organelles. For example, mitochondria handle energy production, while the Golgi apparatus manages protein sorting and shipping.
- Genetic Regulation: The compartmentalization allows for sophisticated regulation of gene expression, leading to cellular differentiation and multicellularity.
- Metabolic Efficiency: Membrane-bound organelles provide distinct environments for different biochemical reactions, enhancing metabolic efficiency and reducing interference between incompatible processes.
- Reproduction and Adaptability: Eukaryotic sexual reproduction introduces genetic variability, enhancing adaptability to changing environments. Prokaryotic rapid asexual reproduction allows for quick population growth under favorable conditions.
Conclusion: The structural differences between prokaryotic and eukaryotic cells underpin their functional distinctions. Prokaryotes, with their simpler structures, are highly adaptable and efficient for rapid growth, making them well-suited for diverse environments. Eukaryotes, with their complex compartmentalization, support specialized functions and multicellular organization, enabling the complexity seen in plants, animals, and fungi.
2. How do mitochondria and chloroplasts exemplify the endosymbiotic theory, and what evidence supports their prokaryotic origins?
Answer:
Endosymbiotic Theory Overview: The endosymbiotic theory posits that mitochondria and chloroplasts originated as free-living prokaryotes that entered into a symbiotic relationship with ancestral eukaryotic cells. Over time, these prokaryotes became permanent organelles within the host cells.
Key Features Supporting Endosymbiotic Theory:
Double Membrane Structure:
- Mitochondria and Chloroplasts: Both organelles possess a double membrane. The outer membrane is similar to the host cell’s membrane, while the inner membrane resembles bacterial membranes, suggesting an engulfing mechanism.
Own Genetic Material:
- Mitochondria and Chloroplasts: Contain their own circular DNA, similar to bacterial genomes, distinct from the linear nuclear DNA of the host eukaryotic cell.
- Genetic Homology: DNA sequences of mitochondria are closely related to those of alpha-proteobacteria, and chloroplast DNA is similar to that of cyanobacteria.
Ribosomes:
- Similarity to Bacterial Ribosomes: Mitochondria and chloroplasts have 70S ribosomes, which are smaller and structurally similar to bacterial ribosomes, unlike the 80S ribosomes found in the eukaryotic cytoplasm.
Reproduction Mechanism:
- Binary Fission: Mitochondria and chloroplasts replicate independently within the cell through binary fission, akin to bacterial reproduction.
Antibiotic Sensitivity:
- Sensitivity to Bacterial Antibiotics: These organelles are affected by antibiotics that target bacterial ribosomes, further indicating their prokaryotic nature.
Phylogenetic Evidence:
- Genetic Sequencing: Comparative analyses show that mitochondrial and chloroplast genes are more similar to those of specific bacteria than to the host eukaryotic genes.
- Evolutionary Trees: Phylogenetic trees place mitochondria and chloroplasts within the bacterial domains, supporting their bacterial origins.
Functional Similarities:
- Energy Conversion: Mitochondria perform cellular respiration, similar to aerobic bacteria, and chloroplasts conduct photosynthesis, akin to photosynthetic bacteria like cyanobacteria.
Presence Across Eukaryotes:
- Universality: All eukaryotic cells possess mitochondria, and photosynthetic eukaryotes also contain chloroplasts, suggesting a common ancestral origin through endosymbiosis.
Consequences and Implications:
- Genetic Integration: Over evolutionary time, many genes from mitochondria and chloroplasts have been transferred to the host nucleus, streamlining the symbiotic relationship.
- Enhanced Cellular Function: The integration of these organelles provided eukaryotic cells with increased energy efficiency and metabolic capabilities, facilitating the evolution of complex multicellular organisms.
Conclusion: Mitochondria and chloroplasts exhibit numerous characteristics that strongly support the endosymbiotic theory, highlighting their origins as free-living prokaryotes that became integral components of eukaryotic cells. This symbiotic relationship has been pivotal in the evolution of complex life forms, demonstrating the profound impact of symbiosis in biological history.
3. Describe the structure and function of the cell membrane, including the roles of its lipid and protein components.
Answer:
Cell Membrane Structure: The cell membrane, also known as the plasma membrane, is a selectively permeable barrier that surrounds the cell, maintaining the internal environment while allowing controlled exchange of substances with the external environment.
Phospholipid Bilayer:
- Composition: Composed primarily of phospholipids, which have hydrophilic (water-attracting) heads and hydrophobic (water-repelling) tails.
- Arrangement: Phospholipids arrange themselves into a bilayer, with hydrophobic tails facing inward and hydrophilic heads facing outward, creating a stable barrier.
- Fluid Mosaic Model: The membrane is dynamic, with lipids and proteins able to move laterally within the layer, allowing flexibility and self-healing.
Lipid Components:
- Phospholipids: Form the basic structure of the membrane, providing fluidity and forming the semi-permeable barrier.
- Cholesterol: Embedded within the bilayer, cholesterol modulates membrane fluidity, making it less fluid at high temperatures and preventing solidification at low temperatures. It also provides structural support.
- Glycolipids: Lipids with carbohydrate groups attached, involved in cell recognition and signaling.
Protein Components:
- Integral (Transmembrane) Proteins:
- Structure: Span the entire membrane, extending from the extracellular side to the cytoplasmic side.
- Functions:
- Transport: Facilitate the movement of molecules across the membrane through channels and carriers (e.g., glucose transporters).
- Receptors: Bind to specific molecules (ligands) and initiate cellular responses (e.g., hormone receptors).
- Enzymes: Catalyze specific biochemical reactions at the membrane surface.
- Peripheral Proteins:
- Structure: Located on the exterior or interior surfaces of the membrane, not spanning the bilayer.
- Functions:
- Support and Structure: Help maintain the cell’s shape and provide a framework for the membrane.
- Signaling: Participate in signal transduction pathways.
- Enzymatic Activity: Some act as enzymes to catalyze reactions near the membrane.
- Glycoproteins and Glycolipids:
- Structure: Proteins or lipids with carbohydrate chains attached.
- Functions: Involved in cell-cell recognition, communication, and adhesion. Important for immune responses and forming the glycocalyx, which protects the cell.
- Integral (Transmembrane) Proteins:
Carbohydrate Components:
- Glycocalyx: A layer of carbohydrates attached to proteins and lipids on the extracellular surface. It plays roles in cell recognition, protection, and interaction with the environment.
Functions of the Cell Membrane:
Selective Permeability:
- Regulation of Substance Movement: Controls the entry and exit of ions, nutrients, waste products, and other molecules, maintaining homeostasis.
- Passive Transport: Includes diffusion and facilitated diffusion, allowing substances to move down their concentration gradients without energy.
- Active Transport: Requires energy (ATP) to move substances against their concentration gradients via pumps (e.g., Na⁺/K⁺-ATPase).
Protection and Structural Support:
- Barrier Function: Protects the cell from physical and chemical damage.
- Anchoring Point: Serves as a scaffold for the cytoskeleton and extracellular matrix, providing structural integrity.
Communication and Signaling:
- Receptors: Integral proteins act as receptors for hormones, neurotransmitters, and other signaling molecules, triggering intracellular responses.
- Signal Transduction: Facilitates the transmission of signals from the extracellular environment to the cell’s interior, enabling cells to respond to changes and coordinate activities.
Cell Adhesion and Interaction:
- Adhesion Molecules: Proteins like cadherins and integrins mediate cell-cell and cell-matrix interactions, essential for forming tissues and maintaining tissue structure.
- Immune Recognition: Glycoproteins on the cell surface help the immune system recognize self from non-self, aiding in defense against pathogens.
Energy Conversion:
- Electron Transport Chain: In mitochondria and chloroplasts, membrane-bound proteins are involved in energy conversion processes like oxidative phosphorylation and photosynthesis.
Conclusion: The cell membrane’s intricate structure, composed of lipids and proteins, enables it to perform a multitude of essential functions. Its selective permeability maintains internal homeostasis, while its proteins facilitate communication, transport, and structural support. Understanding the cell membrane’s structure and functions is fundamental to comprehending cellular interactions, signaling pathways, and the mechanisms underlying various physiological processes.
4. How does the cytoskeleton contribute to cell shape, intracellular transport, and cell division? Discuss the roles of its major components.
Answer:
Cytoskeleton Overview: The cytoskeleton is a dynamic network of protein fibers within the cytoplasm of eukaryotic cells. It provides structural support, maintains cell shape, facilitates intracellular transport, and plays critical roles in cell division and movement.
Major Components of the Cytoskeleton:
Microfilaments (Actin Filaments):
- Structure: Thin (7 nm) helical polymers composed of actin monomers.
- Function:
- Cell Shape and Movement: Support cell shape and enable changes through polymerization and depolymerization. Involved in amoeboid movement, muscle contraction (interacting with myosin), and the formation of cellular extensions like lamellipodia and filopodia.
- Intracellular Transport: Act as tracks for motor proteins (e.g., myosin) to transport vesicles, organelles, and other cargo within the cell.
- Cell Division: Contractile rings composed of actin and myosin facilitate cytokinesis by pinching the cell into two daughter cells.
Intermediate Filaments:
- Structure: Medium (10 nm) sturdy fibers composed of various proteins (e.g., keratins, vimentin, neurofilaments).
- Function:
- Mechanical Stability: Provide tensile strength to resist mechanical stress and maintain cell integrity. Important in cells subjected to stretching or compression, such as epithelial cells and neurons.
- Nuclear Support: Anchor the nucleus and maintain its position within the cell.
- Organizational Framework: Help organize and stabilize the positions of organelles within the cytoplasm.
Microtubules:
- Structure: Thick (25 nm) hollow tubes made of tubulin dimers (α and β tubulin).
- Function:
- Cell Shape and Structure: Provide rigidity and maintain the cell’s overall shape.
- Intracellular Transport: Serve as tracks for motor proteins (kinesin and dynein) to move vesicles, organelles, and other cargo across long distances within the cell.
- Cell Division: Form the mitotic spindle during mitosis and meiosis, ensuring accurate segregation of chromosomes to daughter cells.
- Cilia and Flagella Formation: Constitute the core structure (axoneme) of cilia and flagella, enabling cell movement and fluid movement across cell surfaces.
Roles in Cellular Functions:
Maintaining Cell Shape:
- Structural Support: Microfilaments and intermediate filaments provide the framework that maintains cell shape against internal and external forces.
- Flexibility and Rigidity: Microfilaments offer flexibility for shape changes, while intermediate filaments provide rigidity and resistance to deformation.
Intracellular Transport:
- Vesicle Movement: Microtubules facilitate the directed movement of vesicles and organelles via motor proteins, ensuring proper distribution of cellular components.
- Efficient Logistics: The cytoskeleton organizes intracellular transport pathways, enhancing the efficiency of molecular trafficking within the cell.
Cell Division:
- Mitotic Spindle Formation: Microtubules assemble into the spindle apparatus, which attaches to chromosomes via kinetochores and segregates them to opposite poles.
- Cytokinesis: Microfilaments form a contractile ring that constricts the cell membrane, dividing the cytoplasm and producing two daughter cells.
Cell Movement:
- Amoeboid Movement: Actin polymerization at the leading edge drives pseudopodia formation, enabling cells like amoebae and immune cells to move.
- Flagellar and Ciliary Motion: Microtubule-based structures generate coordinated movements for locomotion and fluid movement across surfaces.
Organizational Roles:
- Organelle Positioning: The cytoskeleton anchors and organizes organelles within the cell, maintaining spatial relationships and facilitating interactions.
- Cell Signaling: Cytoskeletal elements participate in signal transduction pathways, influencing cell behavior and responses to stimuli.
Regulation of the Cytoskeleton:
- Dynamic Assembly and Disassembly: The cytoskeleton constantly undergoes polymerization and depolymerization, allowing rapid reorganization in response to cellular needs.
- Regulatory Proteins: Proteins like actin-binding proteins, tubulin-associated proteins, and motor proteins regulate cytoskeletal dynamics and interactions.
- Signaling Pathways: Cellular signals can modulate cytoskeletal organization through phosphorylation, small GTPases, and other regulatory mechanisms.
Consequences of Cytoskeletal Dysfunction:
- Structural Weakness: Impaired cytoskeletal components can lead to loss of cell shape, reduced mechanical stability, and increased susceptibility to damage.
- Transport Defects: Disruptions in microfilament or microtubule function can hinder intracellular transport, affecting organelle distribution and protein trafficking.
- Division Errors: Faulty spindle formation or contractile ring function can result in unequal chromosome segregation and failed cytokinesis, leading to aneuploidy or multinucleated cells.
- Movement Impairment: Defects in actin or microtubule dynamics can compromise cell motility, impacting processes like wound healing, immune responses, and embryonic development.
Conclusion: The cytoskeleton is essential for maintaining cell structure, facilitating intracellular transport, and ensuring accurate cell division. Its dynamic nature and intricate regulation enable cells to adapt to changing environments and perform complex functions. Understanding the cytoskeleton’s components and roles provides insight into cellular mechanics, development, and the basis of various diseases related to cytoskeletal defects.
5. Explain the process of protein synthesis, detailing the roles of ribosomes, messenger RNA (mRNA), and transfer RNA (tRNA).
Answer:
Protein Synthesis Overview: Protein synthesis is the biological process by which cells build proteins, which are essential for various cellular functions. It involves two main stages: transcription and translation.
Stages of Protein Synthesis:
Transcription (DNA to mRNA):
- Location: Nucleus (in eukaryotes) or cytoplasm (in prokaryotes).
- Process:
- Initiation:
- Promoter Binding: RNA polymerase binds to the promoter region of a gene, signaling the start of transcription.
- DNA Unwinding: The double-stranded DNA helix unwinds, exposing the template strand.
- Elongation:
- RNA Synthesis: RNA polymerase synthesizes a single-stranded mRNA molecule complementary to the DNA template, adding ribonucleotides (A, U, C, G) in the 5’ to 3’ direction.
- Termination:
- Stop Signal: Upon reaching a termination sequence, RNA polymerase detaches, releasing the newly formed pre-mRNA.
- RNA Processing (Eukaryotes):
- 5’ Capping: Addition of a methylated guanine cap for stability and ribosome binding.
- Polyadenylation: Addition of a poly-A tail to protect the mRNA from degradation.
- Splicing: Removal of introns (non-coding regions) and joining of exons (coding regions) to form mature mRNA.
- Initiation:
Translation (mRNA to Protein):
- Location: Ribosomes in the cytoplasm or on the rough endoplasmic reticulum.
- Process:
- Initiation:
- Ribosome Assembly: The small ribosomal subunit binds to the start codon (AUG) on the mRNA.
- tRNA Binding: An initiator tRNA carrying methionine binds to the start codon via its anticodon (UAC).
- Large Subunit Joining: The large ribosomal subunit binds, forming a complete ribosome with A (aminoacyl), P (peptidyl), and E (exit) sites.
- Elongation:
- Codon Recognition: The ribosome reads the next codon on the mRNA.
- tRNA Binding: Corresponding tRNA molecules bring specific amino acids, matching their anticodons to the mRNA codons.
- Peptide Bond Formation: The ribosome catalyzes the formation of peptide bonds between adjacent amino acids, extending the polypeptide chain.
- Translocation: The ribosome moves along the mRNA, shifting the peptidyl-tRNA from the A site to the P site, and freeing the A site for the next tRNA.
- Termination:
- Stop Codon Encounter: When a stop codon (UAA, UAG, UGA) is reached, release factors bind to the ribosome.
- Polypeptide Release: The completed protein is released from the ribosome.
- Ribosome Disassembly: The ribosomal subunits separate, ready to initiate another round of translation.
- Initiation:
Roles of Key Components:
Ribosomes:
- Structure: Composed of rRNA and proteins, ribosomes have two subunits (small and large) that assemble around the mRNA during translation.
- Function: Facilitate the decoding of mRNA into amino acid sequences, catalyze peptide bond formation, and ensure the correct pairing of tRNA anticodons with mRNA codons.
Messenger RNA (mRNA):
- Role: Serves as the template that carries genetic information from DNA to the ribosome, specifying the order of amino acids in a protein.
- Structure: Linear sequence of codons, each consisting of three nucleotides that correspond to specific amino acids or stop signals.
Transfer RNA (tRNA):
- Role: Transport specific amino acids to the ribosome during translation, matching their anticodons with mRNA codons.
- Structure: Cloverleaf shape with an anticodon loop that base-pairs with the mRNA codon and an acceptor stem that binds the corresponding amino acid.
- Function: Ensure the accurate incorporation of amino acids into the growing polypeptide chain by recognizing the correct codon on the mRNA.
Regulation and Accuracy:
- Proofreading: Ribosomes and tRNA molecules have mechanisms to ensure correct codon-anticodon pairing, minimizing errors in protein synthesis.
- Quality Control: Cells have systems to degrade faulty mRNA or misfolded proteins, maintaining protein quality and cellular function.
Conclusion: Protein synthesis is a fundamental cellular process that translates genetic information into functional proteins. Ribosomes, mRNA, and tRNA work in concert to ensure accurate and efficient production of proteins, which are essential for virtually all aspects of cellular structure and function. Understanding this process is crucial for insights into gene expression, regulation, and the molecular basis of various diseases.
6. How do the endoplasmic reticulum (ER) and Golgi apparatus collaborate in the synthesis, modification, and transport of proteins within the cell?
Answer:
Endoplasmic Reticulum (ER) Overview: The endoplasmic reticulum is an extensive network of membranous tubules and flattened sacs within the cytoplasm of eukaryotic cells. It plays a crucial role in the synthesis, folding, modification, and transport of proteins and lipids.
Types of ER:
Rough Endoplasmic Reticulum (RER):
- Structure: Studded with ribosomes on its cytoplasmic surface, giving it a “rough” appearance.
- Function:
- Protein Synthesis: Ribosomes on the RER synthesize proteins destined for secretion, incorporation into membranes, or lysosomes.
- Protein Folding and Quality Control: Chaperone proteins within the RER assist in proper protein folding and ensure quality control by targeting misfolded proteins for degradation.
- Post-Translational Modifications: Proteins undergo modifications such as glycosylation (addition of carbohydrate groups) and formation of disulfide bonds, essential for their stability and function.
Smooth Endoplasmic Reticulum (SER):
- Structure: Lacks ribosomes, giving it a “smooth” appearance.
- Function:
- Lipid Synthesis: Synthesizes lipids, including phospholipids and cholesterol, which are essential components of cellular membranes.
- Detoxification: Metabolizes and detoxifies drugs, toxins, and metabolic waste products, particularly in liver cells.
- Calcium Storage: Stores and regulates intracellular calcium ions, important for various signaling pathways.
- Carbohydrate Metabolism: Involved in processes like gluconeogenesis (production of glucose from non-carbohydrate sources).
Golgi Apparatus Overview: The Golgi apparatus, also known as the Golgi complex or Golgi body, is a series of flattened membranous sacs stacked in a roughly parallel arrangement. It functions as the cell’s post-translational modification and sorting center.
Golgi Apparatus Structure:
- Cisternae: Flattened membrane-bound sacs where protein modification and sorting occur.
- Vesicles: Membrane-bound sacs that transport proteins to and from the ER and Golgi.
Collaboration Between ER and Golgi Apparatus:
Protein Transport from ER to Golgi:
- Vesicle Budding: Proteins synthesized in the RER are packaged into transport vesicles that bud off from the ER membrane.
- Vesicle Movement: Vesicles are transported to the Golgi apparatus, often moving along microtubules with the help of motor proteins.
Protein Modification in the Golgi:
- Glycosylation: Addition and modification of carbohydrate groups on proteins, refining their structure and function.
- Phosphorylation: Addition of phosphate groups to proteins, regulating their activity and signaling functions.
- Sorting and Packaging: Proteins are sorted based on their final destinations (e.g., secretion, lysosomes, plasma membrane) and packaged into specific vesicles.
Vesicle Transport from Golgi to Target Destinations:
- Secretory Pathway: Proteins destined for secretion are packaged into secretory vesicles that fuse with the plasma membrane, releasing their contents outside the cell.
- Membrane Proteins: Proteins destined for the plasma membrane are inserted into vesicles that fuse with the cell membrane, integrating the proteins into the membrane.
- Lysosomal Proteins: Proteins targeted for lysosomes are packaged into vesicles that fuse with late endosomes or directly with lysosomes.
Functional Synergy:
- Efficient Processing: The collaboration ensures that proteins are accurately synthesized, properly folded, and correctly modified before reaching their functional destinations.
- Quality Control: Both the ER and Golgi apparatus participate in quality control, ensuring only properly processed proteins are transported, while misfolded proteins are targeted for degradation.
- Dynamic Response: The ER and Golgi can rapidly respond to changes in cellular demands by adjusting the rate of protein synthesis, modification, and transport.
Examples of Protein Trafficking:
Secretory Proteins:
- Example: Insulin is synthesized in the RER, modified in the Golgi, and packaged into vesicles that secrete it into the bloodstream.
Membrane Proteins:
- Example: Receptor proteins on the cell surface are synthesized in the RER, processed in the Golgi, and inserted into the plasma membrane via vesicle fusion.
Lysosomal Enzymes:
- Example: Digestive enzymes are synthesized in the RER, tagged with mannose-6-phosphate in the Golgi, and transported to lysosomes where they function to break down cellular waste.
Conclusion: The endoplasmic reticulum and Golgi apparatus work in tandem to ensure the efficient synthesis, modification, and distribution of proteins and lipids within the cell. Their coordinated functions are essential for maintaining cellular homeostasis, facilitating communication, and supporting various cellular processes. Understanding their collaboration provides insight into the intricate mechanisms of intracellular transport and protein management.
7. How do lysosomes function in cellular waste management, and what are the consequences of lysosomal dysfunction?
Answer:
Lysosomes Overview: Lysosomes are membrane-bound organelles found in eukaryotic cells, containing a variety of hydrolytic enzymes capable of breaking down all types of biological polymers—proteins, nucleic acids, carbohydrates, and lipids. They play a crucial role in cellular waste management, recycling, and defense.
Structure of Lysosomes:
- Membrane: Encloses the lysosomal enzymes, maintaining an acidic internal environment (pH ~4.5-5.0) optimal for enzyme activity.
- Enzymes: Contain digestive enzymes such as proteases, lipases, nucleases, and carbohydrases.
- Vesicles: Lysosomes can fuse with autophagosomes (containing damaged organelles) and endosomes (containing extracellular materials).
Functions of Lysosomes:
Intracellular Digestion:
- Autophagy: Lysosomes digest and recycle damaged or obsolete organelles and macromolecules within the cell. Autophagosomes engulf the targeted components and fuse with lysosomes, where the contents are broken down and recycled.
- Endocytosis: Lysosomes digest materials ingested from the extracellular environment via endocytosis. Phagosomes (from phagocytosis) and endosomes (from pinocytosis) fuse with lysosomes to degrade their contents.
Waste Removal:
- Breakdown of Cellular Debris: Lysosomes degrade cellular waste products, preventing accumulation of harmful substances and maintaining cellular homeostasis.
Defense Mechanism:
- Pathogen Destruction: In immune cells like macrophages and neutrophils, lysosomes digest engulfed pathogens such as bacteria and viruses, contributing to the body’s defense against infections.
Apoptosis (Programmed Cell Death):
- Caspase Activation: Lysosomal enzymes can be released into the cytoplasm to activate caspases, enzymes that execute apoptosis, aiding in controlled cell death.
Recycling of Cellular Components:
- Reuse of Molecules: Digested materials are transported out of lysosomes and reused for various cellular processes, conserving resources and energy.
Consequences of Lysosomal Dysfunction:
Lysosomal Storage Diseases:
- Definition: Genetic disorders caused by deficiencies in specific lysosomal enzymes, leading to the accumulation of undigested substrates.
- Examples:
- Gaucher Disease: Deficiency in glucocerebrosidase leads to the buildup of glucocerebroside in macrophages.
- Tay-Sachs Disease: Deficiency in hexosaminidase A results in the accumulation of GM2 gangliosides in neurons.
- Pompe Disease: Deficiency in acid alpha-glucosidase causes glycogen accumulation in muscle cells.
- Consequences: Accumulation of substrates disrupts cellular function, leading to organ enlargement, neurological symptoms, and impaired development.
Cellular Damage and Death:
- Accumulation of Waste: Undigested substrates can interfere with cellular processes, causing organelle dysfunction and impaired metabolism.
- Autophagic Blockage: Incomplete autophagy results in the buildup of damaged organelles, contributing to cellular stress and apoptosis.
- Apoptosis Induction: Excessive release of lysosomal enzymes into the cytoplasm can trigger uncontrolled cell death, impacting tissue integrity and function.
Neurodegenerative Diseases:
- Alzheimer’s Disease: Impaired lysosomal function is associated with the accumulation of amyloid-beta plaques and tau tangles.
- Parkinson’s Disease: Dysfunctional lysosomes contribute to the buildup of alpha-synuclein aggregates, affecting neuronal health.
Immune System Impairment:
- Reduced Pathogen Clearance: Defective lysosomes in immune cells hinder the digestion of engulfed pathogens, compromising the body’s ability to fight infections.
Cancer Progression:
- Tumorigenesis: Lysosomal dysfunction can contribute to cancer progression by affecting cellular signaling pathways, apoptosis, and autophagy, enabling cancer cells to evade death and sustain growth.
Mechanisms Preventing Lysosomal Dysfunction:
Proper Enzyme Targeting:
- Mannose-6-Phosphate Tagging: Lysosomal enzymes are tagged with mannose-6-phosphate in the Golgi apparatus, ensuring their delivery to lysosomes via mannose-6-phosphate receptors.
Quality Control Systems:
- ER-Associated Degradation (ERAD): Misfolded lysosomal enzymes are identified and targeted for degradation before they reach lysosomes.
- Chaperone Proteins: Assist in proper folding of lysosomal enzymes, preventing accumulation of non-functional proteins.
Autophagy Regulation:
- Efficient Autophagic Flux: Ensures timely digestion and recycling of cellular components, maintaining lysosomal health and function.
Conclusion: Lysosomes are essential for cellular waste management, recycling, and defense mechanisms. Their dysfunction can lead to severe cellular and systemic consequences, including lysosomal storage diseases, neurodegeneration, immune impairment, and cancer progression. Understanding lysosomal biology is crucial for diagnosing and developing treatments for these conditions, highlighting the organelles’ pivotal role in maintaining cellular and organismal health.
8. How does the cytoplasm facilitate cellular processes, and what roles do organelles play within the cytoplasmic environment?
Answer:
Cytoplasm Overview: The cytoplasm is the gel-like substance within the cell membrane that encompasses all cellular components excluding the nucleus. It consists of the cytosol (a semi-fluid matrix), organelles, cytoskeleton, and various dissolved molecules. The cytoplasm provides a medium for biochemical reactions, structural support, and the movement of organelles and molecules within the cell.
Functions of the Cytoplasm:
Medium for Biochemical Reactions:
- Metabolic Pathways: The cytoplasm houses numerous enzymes and substrates essential for metabolic processes like glycolysis, protein synthesis, and lipid metabolism.
- Homeostasis: Maintains the chemical environment necessary for enzymatic activities, regulating pH, ion concentrations, and osmotic balance.
Structural Support:
- Cell Shape Maintenance: The cytoplasm, in conjunction with the cytoskeleton, helps maintain the cell’s shape and structural integrity.
- Cytoskeletal Anchoring: Provides a scaffold that anchors organelles and facilitates their positioning within the cell.
Intracellular Transport:
- Movement of Organelles and Vesicles: The cytoplasm allows for the movement of organelles and vesicles, often guided by the cytoskeleton and motor proteins.
- Transport of Molecules: Facilitates the diffusion and active transport of molecules to various parts of the cell where they are needed.
Storage of Cellular Components:
- Reservoirs: Stores nutrients, ions, and other molecules that can be quickly mobilized for cellular activities.
- Inclusion Bodies: Accumulate substances like glycogen granules, lipid droplets, and pigment granules, serving as energy reserves or protective pigments.
Roles of Organelles within the Cytoplasmic Environment:
Mitochondria:
- Energy Production: Generate ATP through cellular respiration, providing the energy required for various cellular processes.
- Metabolic Regulation: Involved in the citric acid cycle, fatty acid oxidation, and apoptosis.
Endoplasmic Reticulum (ER):
- Rough ER: Synthesizes proteins destined for secretion or membrane localization, assists in protein folding and quality control.
- Smooth ER: Synthesizes lipids, detoxifies drugs and toxins, stores calcium ions, and participates in carbohydrate metabolism.
Golgi Apparatus:
- Protein Modification and Sorting: Modifies proteins and lipids received from the ER and sorts them for transport to their designated destinations.
- Vesicle Formation: Packages modified molecules into vesicles for delivery within or outside the cell.
Lysosomes:
- Waste Digestion: Contain hydrolytic enzymes that break down macromolecules, damaged organelles, and engulfed pathogens.
- Recycling: Degrade materials and recycle their components for reuse in cellular processes.
Peroxisomes:
- Detoxification: Break down fatty acids and amino acids, detoxify harmful substances through oxidative reactions.
- Reactive Oxygen Species Management: Neutralize reactive oxygen species produced during metabolic processes.
Ribosomes:
- Protein Synthesis: Assemble amino acids into proteins based on mRNA sequences.
- Location: Found free in the cytoplasm or attached to the rough ER, facilitating translation of proteins into the ER lumen or for cytoplasmic use.
Cytoskeleton:
- Structural Framework: Provides support and shape to the cell through microfilaments, intermediate filaments, and microtubules.
- Intracellular Transport: Guides the movement of organelles, vesicles, and molecules via motor proteins like kinesin and dynein.
- Cell Movement and Division: Facilitates processes like cell migration, cytokinesis, and chromosome segregation during cell division.
Vacuoles:
- Storage: Store nutrients, waste products, and other materials. In plant cells, central vacuoles maintain turgor pressure.
- Hydrolytic Functions: In some organisms, vacuoles can digest engulfed particles and pathogens.
Nucleolus:
- Ribosome Biogenesis: Synthesizes and assembles ribosomal RNA (rRNA) with ribosomal proteins to form ribosomal subunits.
Centrosomes and Centrioles:
- Spindle Formation: Organize microtubules during cell division to form the mitotic spindle.
- Cilia and Flagella Assembly: Serve as basal bodies for the growth of cilia and flagella, enabling cell movement.
Dynamic Nature of the Cytoplasm:
- Fluidity: The cytoplasm is not a static environment; it is highly dynamic, allowing organelles and molecules to move, interact, and reorganize in response to cellular needs.
- Signal Integration: Acts as a hub where signaling molecules can diffuse and interact with various targets, integrating cellular responses to external and internal stimuli.
Consequences of Cytoplasmic Dysfunction:
- Metabolic Imbalances: Disruptions in cytoplasmic processes can lead to metabolic disorders, affecting energy production and biosynthesis.
- Structural Instability: Compromised cytoskeletal integrity can result in loss of cell shape, impaired movement, and defective intracellular transport.
- Accumulation of Waste: Inefficient lysosomal function or disrupted vesicular transport can lead to the buildup of waste products, causing cellular toxicity.
Conclusion: The cytoplasm is a vital component of the cell, providing the environment for biochemical reactions, structural support, and the dynamic movement of organelles and molecules. Organelles within the cytoplasm collaborate to ensure efficient cellular functioning, maintenance, and adaptation to changing conditions. Understanding the roles and interactions of cytoplasmic components is essential for comprehending cellular biology and the mechanisms underlying various cellular processes and diseases.
9. What mechanisms regulate the cell cycle, and how do disruptions in these mechanisms contribute to uncontrolled cell proliferation, such as in cancer?
Answer:
Cell Cycle Overview: The cell cycle is the sequence of events that a cell undergoes from one division to the next, encompassing growth, DNA replication, and cell division. It consists of interphase (G₁, S, G₂ phases) and the mitotic phase (mitosis and cytokinesis).
Regulation Mechanisms of the Cell Cycle:
Cyclins and Cyclin-Dependent Kinases (CDKs):
- Cyclins: Regulatory proteins whose levels fluctuate throughout the cell cycle, activating CDKs at specific stages.
- CDKs: Enzymes that, when activated by cyclins, phosphorylate target proteins to drive the cell cycle forward.
- Cyclin-CDK Complexes: Specific complexes (e.g., Cyclin D-CDK4/6, Cyclin E-CDK2, Cyclin A-CDK2, Cyclin B-CDK1) regulate transitions between different phases.
Cell Cycle Checkpoints:
- G₁ Checkpoint (Restriction Point):
- Function: Determines whether the cell has adequate resources, size, and undamaged DNA to proceed to the S phase.
- Regulation: Controlled by the retinoblastoma protein (Rb) and the p53 tumor suppressor. If conditions are unfavorable or DNA is damaged, the checkpoint halts progression.
- G₂ Checkpoint:
- Function: Verifies that DNA replication in the S phase is complete and that there is no DNA damage before entering mitosis.
- Regulation: Involves proteins like p53, which can induce cell cycle arrest or apoptosis if DNA damage is detected.
- M Checkpoint (Spindle Assembly Checkpoint):
- Function: Ensures that all chromosomes are properly attached to the mitotic spindle before anaphase begins.
- Regulation: Proteins like MAD2 and BUBR1 prevent premature separation of sister chromatids, ensuring accurate chromosome segregation.
- G₁ Checkpoint (Restriction Point):
Tumor Suppressor Genes:
- p53:
- Role: Acts as a “guardian of the genome,” initiating cell cycle arrest, DNA repair, or apoptosis in response to DNA damage.
- Impact: Prevents the propagation of cells with genomic abnormalities.
- Rb Protein:
- Role: Regulates the G₁ checkpoint by controlling the progression from G₁ to S phase through interactions with E2F transcription factors.
- Impact: Inhibition of Rb function can lead to uncontrolled cell cycle progression.
- p53:
Oncogenes:
- Definition: Mutated or overexpressed forms of proto-oncogenes that promote cell cycle progression and proliferation.
- Examples: Ras, Myc, and cyclin genes.
- Impact: When activated, oncogenes can drive excessive cell division, contributing to tumor formation.
Apoptosis Pathways:
- Intrinsic Pathway: Triggered by internal cell stress or DNA damage, leading to programmed cell death to eliminate damaged cells.
- Extrinsic Pathway: Initiated by external signals binding to death receptors on the cell surface, also leading to apoptosis.
- Role in Cell Cycle Regulation: Ensures that cells with irreparable damage do not continue to divide.
Disruptions Leading to Uncontrolled Cell Proliferation:
Mutation of Tumor Suppressor Genes:
- Loss of p53 Function: Prevents the cell from halting the cycle in response to DNA damage, allowing accumulation of mutations.
- Rb Dysfunction: Leads to unchecked progression through the G₁ checkpoint, promoting continuous cell division.
Activation of Oncogenes:
- Overexpression or Mutation: Oncogenes can drive excessive proliferation signals, bypassing normal regulatory controls.
- Examples: Mutated Ras can constitutively activate signaling pathways that promote cell growth and division.
Checkpoint Failure:
- Inadequate Control: If checkpoints fail to detect errors or damage, cells with genomic instability continue to divide, increasing the risk of tumorigenesis.
Deregulated Cyclin-CDK Activity:
- Excess Cyclins or CDKs: Overproduction can lead to constant activation of cell cycle progression, irrespective of external or internal signals.
- Inhibition of CDK Inhibitors: Proteins like p21 and p27 that normally inhibit CDKs may be inactivated, removing brakes on the cycle.
Telomere Shortening and Activation of Telomerase:
- Role in Cancer: Normal cells have limited divisions due to telomere shortening. Cancer cells often activate telomerase, maintaining telomere length and allowing indefinite proliferation.
Consequences of Uncontrolled Cell Proliferation:
- Tumor Formation: Accumulation of rapidly dividing cells leads to benign or malignant tumors.
- Metastasis: Cancer cells can invade surrounding tissues and spread to distant organs, complicating treatment.
- Genomic Instability: Continuous division without proper DNA repair increases mutations, driving cancer progression.
- Resistance to Apoptosis: Cancer cells often evade programmed cell death, sustaining their growth despite detrimental changes.
Conclusion: The cell cycle is intricately regulated by cyclins, CDKs, checkpoints, tumor suppressor genes, and oncogenes to ensure accurate and controlled cell division. Disruptions in these regulatory mechanisms can lead to uncontrolled cell proliferation, a hallmark of cancer. Understanding these processes is essential for developing targeted therapies and strategies to prevent and treat cancer.
10. How do cell junctions contribute to tissue integrity and communication, and what are the different types of cell junctions found in animal cells?
Answer:
Cell Junctions Overview: Cell junctions are specialized structures that connect adjacent cells, maintaining tissue integrity, facilitating communication, and regulating the movement of substances between cells. They are essential for the structural and functional coherence of multicellular organisms.
Types of Cell Junctions in Animal Cells:
Tight Junctions (Occluding Junctions):
- Structure: Composed of claudins and occludins that form a seal between adjacent cells near their apical surfaces.
- Function:
- Barrier Formation: Prevent the free passage of molecules and ions through the space between cells, maintaining distinct extracellular environments.
- Apical-Basal Polarity: Help establish and maintain the polarity of epithelial cells by preventing the mixing of apical and basolateral membrane proteins.
- Location: Predominantly found in epithelial tissues lining organs and body cavities (e.g., intestinal epithelium, blood-brain barrier).
Adherens Junctions (Zonula Adherens):
- Structure: Comprised of cadherin proteins linked to the actin cytoskeleton via catenins.
- Function:
- Mechanical Attachment: Provide strong mechanical bonds between cells, maintaining tissue integrity.
- Signal Transduction: Involved in transmitting signals that regulate cell behavior and differentiation.
- Location: Common in epithelial and endothelial tissues, forming a belt-like structure encircling the cell.
Desmosomes (Macula Adherens):
- Structure: Consist of cadherins (desmogleins and desmocollins) linked to intermediate filaments (keratin) via adaptor proteins (desmoplakins and plakoglobins).
- Function:
- Mechanical Strength: Distribute tensile forces across tissues, preventing cells from tearing apart under stress.
- Tissue Integrity: Essential for maintaining the structural integrity of tissues subjected to mechanical stress, such as skin and cardiac muscle.
- Location: Abundant in tissues like the epidermis, heart muscle, and uterus.
Gap Junctions:
- Structure: Composed of connexin proteins that form channels (connexons) allowing direct cytoplasmic communication between adjacent cells.
- Function:
- Electrical and Chemical Coupling: Permit the passage of ions, metabolites, and signaling molecules, enabling coordinated cellular activities.
- Synchronization: Essential for synchronized muscle contractions and coordinated responses in neuronal networks.
- Location: Found in cardiac muscle, smooth muscle, neurons, and various epithelial tissues.
Hemidesmosomes:
- Structure: Similar to desmosomes but connect cells to the extracellular matrix instead of adjacent cells. Composed of integrins linked to intermediate filaments.
- Function:
- Anchoring: Secure epithelial cells to the underlying basement membrane, providing stability and resistance to shear stress.
- Location: Prominently present in epithelial tissues, such as the skin’s basal layer.
Anchoring Junctions:
- Includes: Desmosomes and hemidesmosomes.
- Function: Provide strong adhesion between cells and between cells and the extracellular matrix, ensuring tissue resilience.
Contribution to Tissue Integrity and Communication:
Mechanical Stability:
- Tissue Cohesion: Desmosomes and adherens junctions distribute mechanical forces across tissues, preventing cells from detaching or being damaged under stress.
- Structural Support: Gap junctions and tight junctions help maintain the organized structure of tissues by ensuring cells remain connected and aligned.
Barrier Function:
- Selective Permeability: Tight junctions regulate the movement of substances between cells, maintaining distinct environments on either side of epithelial layers (e.g., preventing leakage in the blood-brain barrier).
- Protection: By forming barriers, cell junctions protect underlying tissues from pathogens and harmful substances.
Cell Communication:
- Direct Cytoplasmic Exchange: Gap junctions allow cells to share ions and small molecules, facilitating coordinated responses and metabolic cooperation.
- Signal Transduction: Adherens junctions participate in signaling pathways that influence cell proliferation, differentiation, and migration.
Cellular Organization:
- Polarity Maintenance: Tight junctions help establish and preserve the polarity of epithelial cells, ensuring directional transport of materials (e.g., nutrient absorption in the intestines).
- Compartmentalization: Junctions contribute to the spatial organization of cells within tissues, essential for specialized functions.
Consequences of Cell Junction Dysfunction:
Loss of Tissue Integrity:
- Detachment and Fragility: Impaired desmosomes or hemidesmosomes can lead to weakened tissue cohesion, making tissues susceptible to injury and detachment (e.g., blistering skin diseases like pemphigus vulgaris).
Barrier Dysfunction:
- Leakage and Inflammation: Disrupted tight junctions can cause leaky barriers, allowing pathogens and toxins to enter tissues and trigger inflammatory responses (e.g., leaky gut syndrome).
Impaired Communication:
- Uncoordinated Activities: Defective gap junctions can lead to desynchronized cellular activities, affecting processes like heart contractions and neural signaling.
Cancer Progression:
- Metastasis: Loss of cell adhesion properties can facilitate cancer cell detachment and spread to other parts of the body.
- Tumor Growth: Impaired signaling through adherens junctions can contribute to uncontrolled cell proliferation.
Conclusion: Cell junctions are fundamental to maintaining the structural integrity, selective permeability, and coordinated function of tissues in animal cells. By providing mechanical stability, regulating substance movement, and facilitating communication, they ensure the proper organization and operation of multicellular organisms. Dysfunction in cell junctions can lead to a range of diseases, highlighting their critical role in health and disease.
11. How do mitochondria and chloroplasts exemplify the endosymbiotic theory, and what evidence supports their prokaryotic origins?
Answer:
Endosymbiotic Theory Overview: The endosymbiotic theory proposes that mitochondria and chloroplasts originated as free-living prokaryotes that entered into a symbiotic relationship with ancestral eukaryotic cells. Over evolutionary time, these prokaryotes became permanent organelles within the host cells.
Key Features Supporting Endosymbiotic Theory:
Double Membrane Structure:
- Mitochondria and Chloroplasts: Both organelles possess a double membrane. The outer membrane resembles the host cell’s membrane, while the inner membrane is similar to bacterial membranes, suggesting an engulfing mechanism.
Own Genetic Material:
- Mitochondria and Chloroplasts: Contain their own circular DNA, similar to bacterial genomes, distinct from the linear nuclear DNA of the host eukaryotic cell.
- Genetic Homology: DNA sequences of mitochondria are closely related to those of alpha-proteobacteria, and chloroplast DNA is similar to that of cyanobacteria.
Ribosomes:
- Similarity to Bacterial Ribosomes: Mitochondria and chloroplasts have 70S ribosomes, which are smaller and structurally similar to bacterial ribosomes, unlike the 80S ribosomes found in the eukaryotic cytoplasm.
Reproduction Mechanism:
- Binary Fission: Mitochondria and chloroplasts replicate independently within the cell through binary fission, akin to bacterial reproduction.
Antibiotic Sensitivity:
- Sensitivity to Bacterial Antibiotics: These organelles are affected by antibiotics that target bacterial ribosomes, further indicating their prokaryotic nature.
Phylogenetic Evidence:
- Genetic Sequencing: Comparative analyses show that mitochondrial and chloroplast genes are more similar to those of specific bacteria than to the host eukaryotic genes.
- Evolutionary Trees: Phylogenetic trees place mitochondria and chloroplasts within the bacterial domains, supporting their bacterial origins.
Functional Similarities:
- Energy Conversion: Mitochondria perform cellular respiration, similar to aerobic bacteria, and chloroplasts conduct photosynthesis, akin to photosynthetic bacteria like cyanobacteria.
Presence Across Eukaryotes:
- Universality: All eukaryotic cells possess mitochondria, and photosynthetic eukaryotes also contain chloroplasts, suggesting a common ancestral origin through endosymbiosis.
Consequences and Implications:
- Genetic Integration: Over evolutionary time, many genes from mitochondria and chloroplasts have been transferred to the host nucleus, streamlining the symbiotic relationship.
- Enhanced Cellular Function: The integration of these organelles provided eukaryotic cells with increased energy efficiency and metabolic capabilities, facilitating the evolution of complex multicellular organisms.
Conclusion: Mitochondria and chloroplasts exhibit numerous characteristics that strongly support the endosymbiotic theory, highlighting their origins as free-living prokaryotes that became integral components of eukaryotic cells. This symbiotic relationship has been pivotal in the evolution of complex life forms, demonstrating the profound impact of symbiosis in biological history.
12. How does apoptosis differ from necrosis, and what are the roles of apoptosis in development and disease?
Answer:
Apoptosis vs. Necrosis Overview: Apoptosis and necrosis are two distinct forms of cell death. While apoptosis is a controlled, programmed process essential for development and homeostasis, necrosis is an uncontrolled, accidental form of cell death often resulting from injury or disease.
Apoptosis:
- Definition: Programmed cell death characterized by an orderly and regulated series of events leading to cell elimination without triggering an inflammatory response.
- Morphological Features:
- Cell Shrinkage: Reduction in cell size.
- Chromatin Condensation: Compaction of nuclear material.
- Membrane Blebbing: Formation of bulges in the plasma membrane.
- Apoptotic Bodies: Fragmentation of the cell into membrane-bound vesicles containing cellular components.
- Biochemical Pathways:
- Intrinsic Pathway: Initiated by internal signals such as DNA damage or oxidative stress, involving mitochondria and the release of cytochrome c, which activates caspases.
- Extrinsic Pathway: Triggered by external signals binding to death receptors on the cell surface, leading to caspase activation.
- Roles in Development and Homeostasis:
- Embryonic Development: Shapes organs and removes unnecessary cells (e.g., digit formation by eliminating interdigital tissue).
- Immune System: Eliminates autoreactive immune cells, preventing autoimmune diseases.
- Tissue Homeostasis: Balances cell proliferation and cell death, maintaining proper cell numbers and function.
Necrosis:
- Definition: Uncontrolled cell death resulting from acute cellular injury, leading to cell lysis and inflammation.
- Morphological Features:
- Cell Swelling: Increased cell volume due to ion and water influx.
- Membrane Rupture: Breakdown of the plasma membrane, releasing cellular contents into the extracellular space.
- Inflammatory Response: Activation of immune cells due to release of intracellular components, which can cause tissue damage.
- Causes of Necrosis:
- Physical Damage: Trauma, extreme temperatures, or mechanical injury.
- Chemical Damage: Toxins, poisons, or exposure to harmful substances.
- Ischemia: Lack of blood supply leading to oxygen and nutrient deprivation.
- Consequences:
- Tissue Inflammation: Release of intracellular contents can provoke inflammation, potentially leading to further tissue damage.
- Loss of Cellular Function: Death of cells disrupts tissue integrity and function.
Roles of Apoptosis in Development and Disease:
Developmental Processes:
- Morphogenesis: Apoptosis shapes tissues and organs during embryonic development (e.g., formation of fingers and toes).
- Neuronal Pruning: Removes excess neurons and strengthens neural networks, essential for proper brain development and function.
Immune System Function:
- Elimination of Autoreactive Cells: Prevents autoimmune responses by removing immune cells that mistakenly target the body’s own tissues.
- Clonal Deletion: Ensures that only beneficial immune cells persist after an immune response.
Tissue Homeostasis:
- Balance of Cell Numbers: Maintains equilibrium between cell proliferation and cell death, ensuring tissues remain healthy and functional.
Cancer:
- Apoptosis Evasion: Cancer cells often develop mechanisms to evade apoptosis, allowing them to survive and proliferate uncontrollably.
- Therapeutic Targets: Many cancer treatments aim to induce apoptosis in cancer cells, restoring the normal cell death processes.
Neurodegenerative Diseases:
- Excessive Apoptosis: Overactivation of apoptotic pathways can lead to the loss of neurons, contributing to diseases like Alzheimer’s, Parkinson’s, and Huntington’s disease.
Autoimmune Diseases:
- Insufficient Apoptosis: Failure to eliminate autoreactive immune cells can result in autoimmune disorders, where the immune system attacks healthy tissues.
Mechanisms Preventing Apoptosis Dysregulation:
Regulatory Proteins:
- Bcl-2 Family: Includes pro-apoptotic and anti-apoptotic proteins that regulate mitochondrial outer membrane permeabilization.
- IAPs (Inhibitor of Apoptosis Proteins): Block caspase activity, preventing apoptosis.
Survival Signals:
- Growth Factors: Promote cell survival by activating pathways that inhibit apoptotic signals.
- PI3K/Akt Pathway: Enhances cell survival by phosphorylating and inactivating pro-apoptotic proteins.
Feedback Mechanisms:
- p53 Tumor Suppressor: Activates apoptotic pathways in response to DNA damage, preventing the propagation of genetically unstable cells.
Consequences of Apoptosis Dysregulation:
- Excessive Apoptosis:
- Degenerative Diseases: Leads to the loss of essential cells and tissues, impairing organ function and contributing to diseases.
- Insufficient Apoptosis:
- Cancer: Allows abnormal cells to survive and accumulate mutations, promoting tumor growth.
- Autoimmune Disorders: Results in the persistence of autoreactive cells that attack the body’s own tissues.
Conclusion: Apoptosis is a vital, regulated process of programmed cell death essential for development, immune function, and maintaining cellular homeostasis. Unlike necrosis, which results from accidental injury and causes inflammation, apoptosis ensures the orderly removal of cells without triggering harmful inflammatory responses. Dysregulation of apoptosis can lead to a range of diseases, including cancer, neurodegenerative disorders, and autoimmune conditions, highlighting its critical role in health and disease.
12. How do cells communicate with each other through signal transduction pathways, and what are the key components involved in these processes?
Answer:
Cell Communication Overview: Cell communication is essential for coordinating cellular activities, maintaining homeostasis, and enabling complex biological processes. Signal transduction pathways facilitate the transmission of signals from the cell’s exterior to its interior, leading to appropriate cellular responses.
Key Components of Signal Transduction Pathways:
Signal Molecules (Ligands):
- Types: Include hormones, neurotransmitters, growth factors, cytokines, and extracellular matrix components.
- Function: Act as messengers that bind to specific receptors on target cells, initiating the signal transduction process.
Receptors:
- Cell Surface Receptors: Located on the plasma membrane, these receptors bind to extracellular ligands. Types include:
- G-Protein Coupled Receptors (GPCRs): Activate intracellular G-proteins upon ligand binding.
- Receptor Tyrosine Kinases (RTKs): Have intrinsic kinase activity that phosphorylates tyrosine residues upon activation.
- Ion Channel Receptors: Open or close ion channels in response to ligand binding, altering ion flow across the membrane.
- Intracellular Receptors: Located within the cytoplasm or nucleus, these receptors bind to lipophilic ligands (e.g., steroid hormones) that can diffuse through the plasma membrane.
- Cell Surface Receptors: Located on the plasma membrane, these receptors bind to extracellular ligands. Types include:
Secondary Messengers:
- Types: Include cyclic AMP (cAMP), inositol triphosphate (IP₃), diacylglycerol (DAG), calcium ions (Ca²⁺), and nitric oxide (NO).
- Function: Amplify and propagate the signal within the cell, transmitting the message from the receptor to target proteins.
- Mechanism: Generated or released in response to receptor activation, activating downstream effectors.
Protein Kinases and Phosphatases:
- Protein Kinases: Enzymes that phosphorylate target proteins, altering their activity, localization, or interactions.
- Protein Phosphatases: Remove phosphate groups from proteins, reversing the actions of kinases.
- Role in Signaling: Regulate the activity of proteins involved in the pathway, controlling the duration and intensity of the signal.
Adaptor Proteins and Scaffold Proteins:
- Adaptor Proteins: Facilitate interactions between different signaling proteins, aiding in the formation of signaling complexes.
- Scaffold Proteins: Organize multiple signaling components into a localized complex, enhancing signal specificity and efficiency.
Transcription Factors:
- Function: Regulate gene expression by binding to specific DNA sequences in the promoter regions of target genes.
- Activation: Often activated by phosphorylation through upstream signaling molecules, allowing them to translocate to the nucleus and influence transcription.
Feedback Mechanisms:
- Negative Feedback: Inhibits signal transduction to prevent overactivation and maintain homeostasis.
- Positive Feedback: Amplifies the signal, driving processes to completion (e.g., blood clotting).
Steps in a Typical Signal Transduction Pathway:
- Signal Reception:
- Ligand Binding: A signal molecule binds to its specific receptor on the cell surface or within the cell.
- Signal Transduction:
- Receptor Activation: Ligand binding induces a conformational change in the receptor, activating its intrinsic activity (e.g., kinase activation).
- Secondary Messenger Generation: Activated receptors stimulate the production or release of secondary messengers, amplifying the signal.
- Cascade of Phosphorylation: Secondary messengers activate a series of protein kinases, creating a phosphorylation cascade that transmits the signal deeper into the cell.
- Cellular Response:
- Activation of Transcription Factors: The cascade often culminates in the activation of transcription factors, altering gene expression and leading to specific cellular responses (e.g., cell growth, differentiation, apoptosis).
- Immediate Cellular Actions: Can also result in rapid changes like ion channel opening, cytoskeletal rearrangement, or enzyme activation.
Examples of Signal Transduction Pathways:
cAMP Pathway:
- Example: Activation of GPCRs by hormones like adrenaline increases cAMP levels via adenylate cyclase, activating protein kinase A (PKA) and leading to metabolic changes.
MAPK/ERK Pathway:
- Example: RTKs activated by growth factors initiate a cascade involving Ras, Raf, MEK, and ERK, ultimately regulating gene expression related to cell proliferation and differentiation.
Calcium Signaling Pathway:
- Example: Binding of neurotransmitters to ion channel receptors releases Ca²⁺, which acts as a secondary messenger to activate various enzymes and facilitate muscle contraction.
Roles in Development and Disease:
Developmental Processes:
- Cell Differentiation: Signal transduction pathways guide stem cells to differentiate into specific cell types, orchestrating tissue and organ formation.
- Morphogenesis: Regulates the spatial and temporal organization of cells during embryonic development.
Immune Responses:
- Cytokine Signaling: Enables immune cells to communicate and coordinate responses to pathogens, inflammation, and tissue injury.
Cancer:
- Oncogenic Signaling: Dysregulation of signal transduction pathways can lead to uncontrolled cell proliferation, survival, and metastasis.
- Therapeutic Targets: Many cancer treatments aim to inhibit aberrant signaling pathways (e.g., RTK inhibitors) to halt tumor growth.
Neurological Disorders:
- Neurotransmitter Signaling: Abnormalities in signal transduction can contribute to diseases like Parkinson’s, Alzheimer’s, and depression.
Metabolic Diseases:
- Insulin Signaling: Defects in insulin signal transduction pathways can lead to diabetes mellitus, affecting glucose uptake and metabolism.
Conclusion: Signal transduction pathways are fundamental to cellular communication, enabling cells to respond to their environment, regulate growth and differentiation, and maintain homeostasis. These pathways involve a complex interplay of receptors, messengers, kinases, and transcription factors, ensuring precise and coordinated cellular responses. Dysregulation of signal transduction can lead to a variety of diseases, underscoring the importance of understanding these mechanisms for developing therapeutic interventions.
Conclusion: These twelve thought-provoking questions and detailed answers cover a wide range of cell biology topics, including cell structure, organelle function, intracellular transport, cell cycle regulation, protein synthesis, apoptosis, and cell communication. They are designed to enhance understanding, promote critical thinking, and reinforce key concepts essential for academic success in cell biology and related STEM disciplines. Utilizing these questions as study aids can help learners grasp the intricate mechanisms that sustain cellular life and contribute to the complexity of multicellular organisms.