Cell Cycle and Division
The cell cycle is a tightly regulated series of events that drives cell growth, replication, and division, enabling organisms to develop, heal, and reproduce. It is central to life processes and intersects multiple biological disciplines. As a foundational concept in science, the study of the cell cycle offers insight into the dynamic nature of living systems. Through understanding the phases and checkpoints of the cycle, students begin to appreciate the balance between cell proliferation and programmed cell death. This subject is a core part of biology and cell biology, where students explore the fundamental units of life and how they coordinate their functions.
The progression through the cell cycle is coordinated by numerous signals, which are studied in depth under cell communication. These cues activate checkpoints to ensure the cell is prepared to proceed through DNA replication and division. Understanding these controls is essential when exploring topics like cell development, cell physiology, and cell structure, as each phase of the cycle reflects specific structural and biochemical changes within the cell.
The evolutionary importance of the cell cycle is evident when considering how it has been conserved across species, tying in with concepts explored in ecology and evolutionary biology. Regulation errors in the cycle can lead to genetic instability or diseases like cancer, making it vital to connect this topic with genetics and subfields like genomics and Mendelian genetics.
On a molecular level, the coordination of the cell cycle is governed by gene expression, mutations, and molecular inheritance—all studied within molecular genetics. Concepts like DNA and RNA interactions, gene expression, and protein synthesis are fundamental to understanding how the cell progresses through its cycle. Errors in these processes, such as genetic mutation, can derail the cycle and have downstream effects on organismal health and reproduction.
The study of molecular basis of inheritance and molecular evolution gives insight into how the cell cycle has been shaped by selective pressures and conserved across lineages. Experimental exploration of the cell cycle often draws upon DNA technology and molecular techniques in research, which allow for precise manipulation and observation of the genetic factors involved in cell division.
Further specialization in population-level patterns like population genetics and quantitative genetics extends understanding of how cell cycle regulation contributes to evolutionary success and variation within species. Additionally, medical innovations stemming from this field are captured in areas such as applications of genetics in medicines, where controlling or correcting cell cycle errors becomes a therapeutic priority.
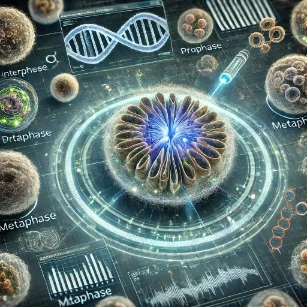
Table of Contents
Overview of the Cell Cycle
The cell cycle is a fundamental process by which cells grow, replicate their genetic material, and divide to produce new cells. It is crucial not only for organismal development, tissue growth, and wound healing, but also for maintaining the balance between cell proliferation and cell death. The cell cycle is typically divided into two main phases:
- Interphase: This is the longest and most metabolically active phase of the cycle. It encompasses cell growth, accumulation of nutrients, and the replication of DNA in preparation for division.
- M Phase: This phase includes both mitosis (or meiosis) and cytokinesis, leading to the physical separation of the cell into two (or four in meiosis) daughter cells.
Interphase occupies approximately 90% of the entire cell cycle, underscoring the complexity and importance of preparing a cell for division. In contrast, the M phase is relatively short but highly orchestrated to ensure accurate distribution of chromosomes. Misregulation of any stage in the cycle can lead to diseases such as cancer, making its precise control a focus of biomedical research. According to the National Center for Biotechnology Information (NCBI), checkpoints within the cycle serve as quality control systems to preserve genomic integrity across generations of cells.
a. Stages of the Cell Cycle
- G1 Phase (Gap 1)
- During G1, the cell increases in size, synthesizes RNA, and produces proteins essential for DNA synthesis. This phase allows the cell to assess whether environmental conditions—such as nutrient levels, temperature, and signaling cues—are favorable for division.
- The G1 checkpoint ensures the cell is ready to proceed to DNA replication. If damage is detected, mechanisms involving p53 and other tumor suppressor proteins can halt the cycle to allow repair or trigger apoptosis.
- G1 is especially important in regulating cell cycle entry, particularly in multicellular organisms where cell proliferation must be tightly controlled.
- S Phase (Synthesis)
- This stage is marked by the complete replication of the cell’s DNA. Each chromosome is duplicated to produce two identical sister chromatids that remain joined at the centromere.
- Histone proteins are synthesized simultaneously to facilitate chromatin packaging, ensuring DNA is compacted and organized correctly for segregation.
- Cells possess mechanisms to detect replication errors, and specialized proteins like DNA polymerase proofreading enzymes play key roles in ensuring high-fidelity replication.
- G2 Phase (Gap 2)
- In G2, the cell undergoes a second growth phase. It prepares for mitosis by synthesizing structural proteins like microtubules and other mitotic machinery.
- DNA is scanned again for any damage missed during the S phase. The G2 checkpoint ensures that the cell will not proceed into mitosis with unreplicated or damaged DNA.
- Cells that fail this checkpoint may enter a quiescent state (G0) or activate apoptosis pathways. G2 is also responsive to stress signals such as oxidative damage and nutrient deprivation.
- M Phase (Mitosis or Meiosis)
- This is the division phase, where the duplicated genome is separated into new cells. Mitosis produces two identical diploid daughter cells and is essential for tissue growth, maintenance, and repair.
- Meiosis, on the other hand, involves two successive divisions and results in four non-identical haploid gametes, crucial for genetic diversity in sexually reproducing organisms.
- M phase is further subdivided into prophase, metaphase, anaphase, and telophase, followed by cytokinesis. Each sub-stage plays a critical role in ensuring accurate chromosome alignment and segregation.
- The spindle assembly checkpoint (SAC) during metaphase ensures that all chromosomes are correctly attached to spindle fibers before separation occurs. Defects in this checkpoint can lead to aneuploidy and various developmental disorders.
Regulation of the Cell Cycle
The regulation of the cell cycle is a highly intricate and finely balanced system that ensures cells only divide under appropriate conditions. It involves multiple layers of control, integrating internal metabolic states and external environmental cues to maintain genomic integrity and cellular function. Disruption in these regulatory mechanisms is a hallmark of numerous diseases, particularly cancer. The primary components orchestrating this control are cyclins, cyclin-dependent kinases (CDKs), checkpoints, tumor suppressor genes, and oncogenes, which work in tandem to enforce the correct timing, sequence, and fidelity of cell cycle events.
a. Cyclins and Cyclin-Dependent Kinases (CDKs)
- Cyclins are proteins whose concentrations fluctuate in a cyclic fashion throughout the cell cycle. They act as molecular clocks, determining the progression from one phase to the next. They exert their function by activating cyclin-dependent kinases (CDKs), which are serine/threonine kinases that phosphorylate specific substrates to initiate various cell cycle processes.
- CDKs are present in the cell at relatively constant levels but remain inactive unless bound by their partner cyclins. Once activated, CDK-cyclin complexes target numerous proteins that control DNA replication, chromosome condensation, and mitotic entry.
- Each cyclin-CDK complex is responsible for a particular phase transition:
- G1/S checkpoint: Cyclin D-CDK4/6 facilitates the progression through G1 by phosphorylating the retinoblastoma (RB) protein, thereby freeing transcription factor E2F to activate genes required for S phase. Cyclin E-CDK2 further prepares the cell for DNA synthesis.
- S Phase: Cyclin A-CDK2 plays a crucial role in initiating and maintaining DNA replication.
- G2/M checkpoint: Cyclin B-CDK1, also known as the maturation-promoting factor (MPF), triggers the onset of mitosis by phosphorylating proteins involved in chromatin condensation and spindle formation.
- The sequential activation and inactivation of these complexes are regulated by feedback loops and proteolytic degradation of cyclins via the ubiquitin-proteasome pathway. Dysregulation in cyclin or CDK expression has been implicated in various cancers and proliferative disorders.
b. Checkpoints in the Cell Cycle
Checkpoints act as surveillance mechanisms to monitor the integrity of critical cell cycle events. These points act as decision gates where the cell evaluates whether to proceed, pause for repair, or exit the cycle. This checkpoint system is especially vital in preventing the transmission of damaged or incomplete genetic material.
- G1 Checkpoint (Restriction Point): This is the first major checkpoint and determines whether the cell commits to division. It assesses cell size, nutrient status, DNA integrity, and extracellular signals. Cells that do not meet the requirements may enter a quiescent state (G0) or undergo programmed cell death.
- G2 Checkpoint: Functions after DNA synthesis, ensuring the genome has been faithfully replicated. It halts progression if there are double-stranded breaks or replication errors. Repair proteins and delay mechanisms are activated to maintain genome fidelity.
- Metaphase Checkpoint (Spindle Assembly Checkpoint): Ensures that all chromosomes are aligned at the metaphase plate and are correctly attached to spindle microtubules. Defective attachment or tension triggers a block in anaphase onset, preventing chromosomal missegregation and aneuploidy.
These checkpoints are crucial in preventing uncontrolled proliferation and maintaining genetic stability. For a more detailed discussion of checkpoint control mechanisms and their implications in cancer biology, the Nature Scitable database offers an excellent educational overview.
c. Role of Tumor Suppressors and Oncogenes
- Tumor Suppressors: These are genes that function to inhibit cell cycle progression, promote DNA repair, or induce apoptosis under stress. Key examples include:
- p53: Known as the “guardian of the genome,” this protein halts the cycle in response to DNA damage by activating p21, a CDK inhibitor. It can also initiate programmed cell death if the damage is irreparable. Mutations in p53 are found in more than 50% of human cancers.
- RB (Retinoblastoma protein): Acts as a gatekeeper in G1 by sequestering transcription factors like E2F. When phosphorylated by CDKs, RB releases E2F, allowing cell cycle progression. Loss-of-function mutations in RB result in unchecked cell division.
- Oncogenes: These are mutated or overexpressed versions of normal proto-oncogenes that promote cell proliferation. When activated inappropriately, they override normal checkpoint controls. Examples include:
- Ras: A small GTPase involved in transmitting signals from growth factor receptors. Mutant Ras proteins remain constitutively active, driving cells into continuous division.
- Myc: A transcription factor that promotes expression of genes involved in growth and metabolism. Amplification of Myc is associated with aggressive tumors.
- The balance between tumor suppressor activity and oncogene activation determines whether a cell maintains homeostasis or transitions into a neoplastic state. Modern cancer therapies often target these regulatory pathways to halt tumor progression.
Mitosis: Division for Growth and Repair
Mitosis is a tightly regulated process of nuclear division in eukaryotic cells that ensures the equal distribution of replicated chromosomes into two daughter cells. This mechanism is vital for various biological functions such as embryonic development, tissue regeneration, wound healing, and the maintenance of stem cell populations. The process results in the formation of two genetically identical diploid cells from a single diploid parent, preserving chromosomal integrity across generations of cells. Mitosis also plays a critical role in asexual reproduction for unicellular organisms like yeast and certain multicellular organisms such as hydra.
The fidelity of mitosis is crucial because errors in chromosome segregation can lead to aneuploidy, a hallmark of many cancers and developmental disorders. Therefore, the mitotic process is orchestrated through a series of carefully timed and controlled stages involving dynamic changes in chromosome structure, spindle apparatus assembly, and nuclear envelope remodeling.
Stages of Mitosis
Mitosis is classically divided into five stages: prophase, metaphase, anaphase, telophase, and cytokinesis. Each stage is marked by distinct morphological and molecular changes that ensure the accurate partitioning of genetic material.
- Prophase
- Chromatin Condensation: The long strands of chromatin compact into discrete, visible chromosomes, each consisting of two sister chromatids joined at a centromere. This compaction is facilitated by condensin proteins and topoisomerases.
- Nucleolar Disassembly and Nuclear Envelope Breakdown: The nucleolus disappears, and the nuclear envelope begins to disintegrate to allow spindle fibers access to the chromosomes. Phosphorylation of nuclear lamins by CDKs drives this envelope breakdown.
- Mitotic Spindle Formation: Microtubules extend from centrosomes, which migrate to opposite poles of the cell. In animal cells, centrioles play a role in organizing the spindle apparatus. The spindle ensures that chromatids are evenly distributed during division.
- Metaphase
- Chromosome Alignment: All chromosomes are guided by spindle microtubules to align at the cell’s equatorial plane, forming the metaphase plate. Proper alignment is critical for equal segregation.
- Spindle Attachment: Each sister chromatid pair is attached to opposite spindle poles via kinetochores, which are protein complexes at the centromeres. The metaphase checkpoint ensures all chromosomes are properly attached before progression.
- Anaphase
- Chromatid Separation: Cohesin proteins that hold sister chromatids together are cleaved by separase, enabling the chromatids to separate.
- Movement to Poles: The separated chromatids (now individual chromosomes) are pulled to opposite poles by the shortening of kinetochore microtubules and elongation of the spindle poles. This ensures each daughter cell receives an identical set of chromosomes.
- Telophase
- Chromosome Decondensation: The chromosomes begin to uncoil and return to their less compact chromatin state, becoming less visible under the microscope.
- Reformation of Nuclear Envelopes: New nuclear envelopes form around each set of chromosomes, resulting in two nuclei in one cell. This marks the end of nuclear division.
- Nucleoli Reappear: Nucleolar components reassemble, indicating a return to a transcriptionally active state in each nucleus.
- Cytokinesis
- Division of the Cytoplasm: This final step physically separates the cell into two. It begins during telophase and completes shortly after mitosis.
- Animal Cells: A contractile ring of actin filaments forms beneath the plasma membrane at the equator of the cell, creating a cleavage furrow that deepens until the cell pinches in two.
- Plant Cells: Due to the rigid cell wall, a cell plate forms from vesicles originating in the Golgi apparatus. These vesicles fuse at the center of the cell and grow outward, eventually forming a new cell wall that separates the daughter cells.
- For an in-depth animation of these stages, the Cells Alive interactive mitosis animation provides an excellent visual walkthrough.
Throughout mitosis, cells rely on accurate timing and the function of motor proteins, microtubules, and numerous regulatory enzymes. Any disruption in the progression through these stages—such as premature anaphase entry or spindle fiber defects—can result in chromosomal instability, contributing to tumorigenesis or developmental anomalies. Understanding mitosis not only deepens our grasp of cell biology but also informs targeted therapies in oncology, where certain drugs (e.g., taxanes and vinca alkaloids) disrupt mitotic spindles to inhibit cancer cell proliferation.
Meiosis: Division for Sexual Reproduction
Meiosis is a fundamental biological process that enables sexual reproduction in eukaryotic organisms. It ensures the transmission of genetic material from parent to offspring while introducing genetic diversity, a cornerstone of evolution and species adaptability. Unlike mitosis, which maintains the diploid chromosome number, meiosis results in the formation of four genetically distinct haploid cells (gametes), each containing half the number of chromosomes as the original cell. This halving is crucial so that upon fertilization, the zygote restores the diploid chromosome number, preserving species-specific genomic integrity.
Meiosis occurs in two successive stages: Meiosis I, a reductional division that separates homologous chromosomes, and Meiosis II, an equational division that separates sister chromatids. Both divisions are tightly regulated and involve precise chromosomal movements and recombination events that contribute to genetic diversity. Meiosis is particularly significant in germ cells—sperm in males and eggs in females—and is integral to heredity, population variability, and adaptation.
Stages of Meiosis
The process of meiosis comprises two consecutive divisions without an intervening round of DNA replication. These stages are as follows:
- Meiosis I: Reduction Division
This phase reduces the chromosome number from diploid (2n) to haploid (n) and is characterized by pairing and segregation of homologous chromosomes.
- Prophase I: This is the longest and most complex stage of meiosis, subdivided into five substages—leptotene, zygotene, pachytene, diplotene, and diakinesis.
- Homologous chromosomes undergo synapsis, forming tetrads through the synaptonemal complex.
- Crossing over occurs, where genetic material is exchanged between non-sister chromatids at structures called chiasmata. This recombination increases genetic variability in offspring.
- Nuclear envelope dissolves and spindle fibers begin to form.
- Metaphase I: Homologous chromosome pairs align along the metaphase plate.
- Each homolog is attached to spindle fibers from opposite poles, ensuring their segregation.
- Independent assortment occurs here—maternal and paternal chromosomes align randomly—further enhancing genetic diversity.
- Anaphase I: Homologous chromosomes are pulled to opposite poles.
- Sister chromatids remain joined at the centromere, unlike mitosis where they separate.
- Telophase I and Cytokinesis: Two haploid cells are formed.
- Each cell contains half the original chromosome number, with chromosomes still consisting of two sister chromatids.
- In some species, the nuclear envelope briefly reforms; in others, cells proceed directly to Meiosis II.
- Prophase I: This is the longest and most complex stage of meiosis, subdivided into five substages—leptotene, zygotene, pachytene, diplotene, and diakinesis.
- Meiosis II: Equational Division
This phase closely resembles mitosis, separating the sister chromatids of each chromosome.
- Prophase II: Chromosomes condense again, and new spindle apparatus forms in each haploid cell.
- Metaphase II: Chromosomes align individually along the metaphase plate.
- Anaphase II: Sister chromatids finally separate and are pulled to opposite poles.
- Telophase II and Cytokinesis: Four genetically distinct haploid daughter cells are formed.
- These cells mature into gametes—sperm or egg cells—depending on the organism’s sex.
Meiosis plays an essential role in evolutionary biology, enabling genetic recombination and variability. It is the biological mechanism that underlies phenomena such as Mendelian inheritance, genetic linkage, and chromosomal disorders like Down syndrome (trisomy 21), which result from nondisjunction during meiotic divisions. Understanding meiosis is also foundational in reproductive medicine, genetic engineering, and developmental biology. For a detailed interactive guide to meiosis with animations and quizzes, visit the Nature Education Scitable resource.
Abnormalities in the Cell Cycle and Their Role in Disease
The cell cycle is a tightly regulated series of events that ensure accurate DNA replication and equal distribution of genetic material into daughter cells. When the regulatory mechanisms of the cell cycle fail, cells may divide uncontrollably or accumulate genetic damage, leading to a host of pathological conditions, most notably cancer. These abnormalities arise due to mutations in key regulatory genes, chromosomal missegregation, or environmental influences that disrupt the balance between cell proliferation and cell death.
One of the most profound consequences of cell cycle dysregulation is the development of cancer. Cancer occurs when cells acquire mutations that allow them to bypass normal checkpoints, avoid apoptosis, and proliferate indefinitely. These mutations often target two major classes of genes: oncogenes and tumor suppressor genes.
- Oncogenes: These are mutated or overexpressed versions of normal genes called proto-oncogenes, which typically promote cell growth and division in a controlled manner.
- When mutated, oncogenes can cause the cell to divide uncontrollably even in the absence of growth signals.
- Examples include the Ras gene, which, when mutated, leads to continuous activation of growth pathways, and HER2, which is overexpressed in certain breast cancers.
- Tumor Suppressor Genes: These genes encode proteins that inhibit cell division, repair damaged DNA, or initiate apoptosis.
- When these genes are inactivated or deleted, the protective checkpoints are removed, allowing damaged or abnormal cells to continue dividing.
- Notable examples include p53, known as the “guardian of the genome,” and RB, which prevents premature progression from G1 to S phase.
Mutations in these key regulators disturb the normal checks and balances of the cell cycle, leading to genomic instability and tumorigenesis. Inherited mutations in these genes significantly increase cancer risk. For instance:
- Retinoblastoma: A rare eye cancer primarily affecting children, caused by mutations in the RB1 gene, which normally inhibits E2F transcription factors and prevents excessive entry into S phase. When both alleles of RB1 are inactivated, cells bypass the G1 checkpoint unchecked.
- Li-Fraumeni Syndrome: A hereditary disorder caused by germline mutations in the TP53 gene, resulting in a predisposition to a wide range of cancers including sarcomas, breast cancer, brain tumors, and leukemia. Individuals inherit a defective copy of the p53 gene, impairing their ability to initiate apoptosis in response to DNA damage.
In addition to cancer, errors during cell division can lead to chromosomal abnormalities, which have severe developmental and physiological consequences:
- Down Syndrome (Trisomy 21): Results from nondisjunction during meiosis, where chromosome 21 fails to segregate properly, leading to an extra copy in the resulting gamete. Affected individuals exhibit intellectual disabilities, characteristic facial features, and increased risk of congenital heart disease.
- Klinefelter Syndrome (XXY) and Turner Syndrome (XO): Caused by nondisjunction of sex chromosomes, these syndromes affect sexual development and fertility.
Disruption of the cell cycle can also contribute to neurodegenerative diseases. For example, neurons are typically in a permanent G0 phase (non-dividing state), but in Alzheimer’s disease, aberrant re-entry into the cell cycle has been observed, which may contribute to neuronal death. Furthermore, improper cell cycle control plays a role in tissue degeneration, chronic inflammation, and impaired wound healing.
Recent research in cell cycle-related diseases has led to the development of targeted therapies. For example, CDK4/6 inhibitors such as palbociclib are used to treat hormone receptor-positive breast cancer by halting the cell cycle in G1 phase. Such treatments underscore the therapeutic value of understanding the molecular mechanisms underlying cell cycle dysregulation. For a comprehensive review on the link between cell cycle regulation and cancer therapies, refer to this review published in the International Journal of Molecular Sciences.
In conclusion, the cell cycle is not just a routine cellular process—it is a tightly orchestrated sequence that maintains organismal health. Abnormalities in its regulation can result in devastating diseases, making it a focal point of both basic research and clinical innovation. By unraveling the molecular basis of cell cycle control, scientists and clinicians can devise new strategies to detect, prevent, and treat a wide spectrum of disorders linked to cellular proliferation and genetic integrity.
Why Study Cell Cycle
Fundamentals of Cell Reproduction
The cell cycle describes how cells grow, replicate their DNA, and divide. This is essential for understanding growth, tissue repair, and reproduction. Mastery of these processes lays the groundwork for studying biology at cellular and organismal levels.
Insights into Cancer Biology
Cancer results from uncontrolled cell division due to mutations in cell cycle regulation. By studying the cell cycle, students learn how normal regulation is disrupted in diseases. This knowledge is key to developing therapies and preventive strategies.
Applications in Medicine and Therapy
Many treatments, such as chemotherapy and radiation, target specific phases of the cell cycle. Understanding these phases helps in designing more effective and less harmful therapies. It also aids in the timing of treatment protocols for better outcomes.
Biotechnological and Agricultural Relevance
Manipulating the cell cycle is useful in plant breeding, cloning, and tissue culture techniques. It also plays a role in producing genetically modified organisms. Students can apply this knowledge across both medical and agricultural biotechnology sectors.
Preparation for Cell and Molecular Research
Knowledge of the cell cycle is foundational for advanced research in molecular biology, developmental biology, and genetics. It prepares students to engage in experimental work involving DNA replication, mitosis, and cell signaling. This is crucial for academic and research careers.
Conclusion
The cell cycle and its associated processes of mitosis and meiosis represent one of the most fundamental and tightly controlled systems in biology. These mechanisms are vital not only for the development and maintenance of multicellular organisms but also for maintaining the fidelity of genetic information through successive generations. Every phase of the cell cycle is intricately monitored and regulated through an elaborate network of signaling molecules, enzymes, and checkpoint controls to prevent errors that could threaten cellular integrity or organismal viability.
During normal cellular activity, progression through the cell cycle allows organisms to grow, replace damaged tissues, and reproduce. Interphase enables the cell to grow and replicate its DNA, while mitosis ensures equal segregation of chromosomes into daughter cells. In contrast, meiosis allows for genetic diversity and the production of gametes, ensuring that sexual reproduction results in varied and adaptable offspring. These processes work in perfect synchrony to maintain tissue homeostasis and promote survival in changing environments.
However, when the regulation of this cycle fails, the consequences can be profound. A breakdown in checkpoint fidelity or aberrant expression of cyclins and CDKs can lead to genomic instability, unrestrained proliferation, and ultimately cancer. Mutations in tumor suppressor genes such as p53 or RB remove vital control barriers, allowing cells to bypass critical checkpoints and continue dividing despite the presence of DNA damage. Similarly, the activation of oncogenes like Ras or Myc can push cells into relentless replication, independent of external growth cues.
Diseases like cancer, congenital chromosomal syndromes, and certain neurological disorders can all be traced back to disturbances in cell cycle regulation. For example, trisomy 21 (Down syndrome) results from faulty chromosome segregation during meiosis, while aggressive tumors often arise from accumulated mitotic errors. In such cases, the absence of proper regulatory oversight transforms what should be a well-orchestrated process into a driver of pathological change.
Understanding the molecular mechanisms governing the cell cycle has catalyzed revolutionary breakthroughs in medical science. The development of targeted therapies, such as CDK inhibitors, exemplifies how fundamental cell biology can be translated into effective treatments for patients with specific genetic mutations. For instance, the introduction of CDK4/6 inhibitors like palbociclib in the treatment of breast cancer has proven effective in slowing tumor growth by arresting cells in the G1 phase of the cell cycle.
Additionally, ongoing research into the interaction between the cell cycle and the immune system, metabolism, and aging processes opens new frontiers in personalized medicine. Therapies designed to reactivate damaged checkpoints, induce selective apoptosis in abnormal cells, or modify cell cycle kinetics are showing promise in clinical trials for a range of diseases, from cancer to neurodegenerative conditions. Resources such as this Nature collection on cell cycle research offer valuable insights into how this field continues to evolve.
In summary, the cell cycle is not just a mechanistic routine—it is the guardian of cellular and organismal continuity. Its precision safeguards life, and its failure signals disease. By deepening our understanding of how cells grow, divide, and die, researchers are better equipped to design strategies that not only treat illness but also restore the natural balance of life at its most fundamental level. For students, scientists, and clinicians alike, the study of the cell cycle remains a cornerstone of biological and biomedical knowledge, with enduring relevance to both academic inquiry and therapeutic innovation.
Cell Cycle and Division: Review Questions with Detailed Answers
Q1. What are the distinct phases of the cell cycle, and what key processes occur in each phase?
Answer:
The cell cycle is a series of ordered events that lead to cell division and duplication. It consists of two main stages: Interphase and Mitotic (M) Phase, each further divided into specific phases.
1. Interphase:
G₁ Phase (Gap 1):
- Processes:
- Cell growth and enlargement.
- Synthesis of mRNA and proteins necessary for DNA replication.
- Preparation for DNA synthesis.
- Significance: Ensures the cell has adequate size, nutrients, and energy to proceed to DNA replication.
- Processes:
S Phase (Synthesis):
- Processes:
- DNA replication occurs, doubling the genetic material.
- Duplication of centrosomes.
- Significance: Ensures each daughter cell receives an identical set of chromosomes.
- Processes:
G₂ Phase (Gap 2):
- Processes:
- Further cell growth and protein synthesis.
- Production of microtubules for mitosis.
- Preparation for mitosis.
- Significance: Finalizes cell growth and prepares the cell for mitotic division.
- Processes:
2. Mitotic (M) Phase:
- Mitosis:
- Prophase:
- Chromatin condenses into visible chromosomes.
- Nuclear envelope begins to disintegrate.
- Formation of the mitotic spindle begins.
- Metaphase:
- Chromosomes align at the cell’s equatorial plate.
- Spindle fibers attach to the centromeres of chromosomes.
- Anaphase:
- Sister chromatids are pulled apart to opposite poles of the cell.
- Telophase:
- Chromosomes decondense back into chromatin.
- Nuclear envelopes reform around each set of chromosomes.
- Prophase:
- Cytokinesis:
- Processes:
- Division of the cytoplasm into two daughter cells.
- Formation of a cleavage furrow in animal cells or a cell plate in plant cells.
- Significance: Results in two genetically identical daughter cells, each with its own nucleus and cytoplasm.
- Processes:
Summary of Key Processes:
- Interphase: Growth, DNA replication, and preparation for division.
- M Phase: Chromosome segregation and cytoplasmic division.
Conclusion: Understanding the distinct phases of the cell cycle is fundamental to comprehending how cells grow, replicate their DNA, and divide. Each phase is meticulously regulated to ensure accurate and efficient cell division, maintaining genetic stability across generations.
Q2. How do cyclins and cyclin-dependent kinases (CDKs) regulate the progression of the cell cycle?
Answer:
Cyclins and Cyclin-Dependent Kinases (CDKs):
Cyclins and CDKs are crucial regulatory proteins that control the progression of the cell cycle. Their interaction ensures that the cell cycle proceeds in an orderly and timely manner, coordinating various cellular processes required for cell division.
1. Cyclins:
- Definition: Regulatory proteins whose levels fluctuate throughout the cell cycle.
- Function: Activate CDKs by binding to them.
- Types:
- Cyclin D: Active during G₁ phase.
- Cyclin E: Active at the G₁/S transition.
- Cyclin A: Active during S and G₂ phases.
- Cyclin B: Active during G₂/M transition.
2. Cyclin-Dependent Kinases (CDKs):
- Definition: Serine/threonine kinases that, when activated by cyclins, phosphorylate target proteins to drive cell cycle progression.
- Function: Catalyze the phosphorylation of specific substrates, initiating key events in the cell cycle.
3. Regulation Mechanism:
- Activation:
- Cyclin Synthesis: Cyclin levels increase at specific cell cycle phases.
- Cyclin-CDK Binding: Cyclins bind to their corresponding CDKs, activating the kinase.
- Phosphorylation of Targets: Activated CDKs phosphorylate proteins that promote progression to the next phase.
- Inhibition:
- CDK Inhibitors (CKIs): Proteins like p21, p27 bind to cyclin-CDK complexes, inhibiting their activity.
- Degradation of Cyclins: Proteasomes degrade cyclins after they have fulfilled their role, leading to inactivation of CDKs.
- Feedback Loops: Positive and negative feedback mechanisms ensure proper timing and order of cell cycle events.
4. Specific Examples:
- G₁ to S Transition:
- Cyclin D-CDK4/6: Phosphorylates the retinoblastoma protein (Rb), releasing E2F transcription factors.
- E2F Activation: Promotes expression of genes required for DNA replication.
- S Phase Progression:
- Cyclin A-CDK2: Initiates DNA replication by activating replication machinery.
- G₂ to M Transition:
- Cyclin B-CDK1: Triggers the onset of mitosis by phosphorylating proteins involved in chromosome condensation and spindle formation.
5. Importance of Cyclin-CDK Regulation:
- Ensures Orderly Progression: Prevents the cell from entering a new phase before completing the current one.
- Prevents Uncontrolled Division: Dysregulation can lead to unchecked cell proliferation, contributing to cancer development.
- Facilitates Checkpoint Responses: Integrates signals from cell cycle checkpoints to halt progression if errors are detected.
Conclusion: Cyclins and CDKs form a tightly regulated system that governs the cell cycle’s progression. By controlling the activation and inactivation of CDKs through cyclin binding and degradation, cells ensure that division occurs accurately and in response to internal and external cues. This regulation is essential for maintaining genomic integrity and preventing diseases such as cancer.
Q3. What are the key checkpoints in the cell cycle, and how do they ensure the fidelity of cell division?
Answer:
Key Checkpoints in the Cell Cycle:
Cell cycle checkpoints are surveillance mechanisms that monitor the integrity of the cell’s DNA and the proper completion of critical processes before allowing progression to the next phase. They ensure that cells do not pass on damaged or incomplete genetic material, maintaining genomic stability.
1. G₁ Checkpoint (Restriction Point):
- Location: Between G₁ phase and S phase.
- Function: Determines whether the cell has sufficient size, nutrients, and energy to proceed with DNA replication.
- Key Factors Monitored:
- DNA Integrity: Detects DNA damage.
- External Signals: Growth factors and environmental conditions.
- Cell Size and Metabolic Status: Ensures adequate resources for division.
- Regulation:
- Retinoblastoma Protein (Rb): Phosphorylation by Cyclin D-CDK4/6 releases E2F transcription factors, promoting S phase entry.
- p53 Tumor Suppressor: Activated in response to DNA damage, inducing expression of p21 CKI, which inhibits Cyclin-CDK complexes, halting the cell cycle.
2. G₂ Checkpoint:
- Location: Between G₂ phase and M phase.
- Function: Ensures that DNA replication in S phase has been accurately completed without errors.
- Key Factors Monitored:
- DNA Integrity: Detects DNA damage or incomplete replication.
- Replication Completion: Confirms that all chromosomes are fully replicated.
- Regulation:
- ATM/ATR Kinases: Detect DNA damage and activate p53 or other effectors.
- Cyclin B-CDK1 Inhibition: CKIs like p21 inhibit Cyclin B-CDK1, preventing entry into mitosis until issues are resolved.
3. Metaphase Checkpoint (Spindle Assembly Checkpoint):
- Location: During metaphase of mitosis.
- Function: Ensures that all chromosomes are properly attached to the mitotic spindle before anaphase begins.
- Key Factors Monitored:
- Chromosome Alignment: All chromosomes must be aligned at the metaphase plate.
- Spindle Attachment: Kinetochores of chromosomes must be attached to spindle fibers from opposite poles.
- Regulation:
- MAD and BUB Proteins: Inhibit the anaphase-promoting complex/cyclosome (APC/C) until all chromosomes are correctly attached.
- APC/C Activation: Once all chromosomes are properly attached, APC/C triggers the separation of sister chromatids and progression to anaphase.
4. Anaphase Checkpoint:
- Location: Transition from metaphase to anaphase.
- Function: Confirms that all sister chromatids are correctly attached and aligned before their separation.
- Key Factors Monitored:
- Kinetochore-Microtubule Attachment: Ensures tension is applied to chromosomes, indicating correct attachment.
- Regulation:
- MAD2: Prevents APC/C activation until all sister chromatids are ready for separation.
5. G₀ Phase:
- Definition: A resting state where cells exit the cell cycle.
- Function: Allows cells to perform specialized functions without dividing.
- Re-entry Conditions: Cells can re-enter the cell cycle from G₀ in response to specific signals.
Mechanisms Ensuring Fidelity:
- DNA Repair Pathways: Activated at checkpoints to fix detected DNA damage before progression.
- Cell Cycle Arrest: Temporarily halts the cell cycle to allow time for repairs.
- Apoptosis Induction: If damage is irreparable, checkpoints can trigger programmed cell death to prevent propagation of mutations.
Consequences of Checkpoint Failures:
- Genomic Instability: Leads to mutations, chromosomal aberrations, and aneuploidy.
- Cancer Development: Checkpoint failures can result in uncontrolled cell division and tumor formation.
- Cell Death or Senescence: Severe checkpoint activation can lead to apoptosis or permanent cell cycle exit.
Conclusion: Cell cycle checkpoints are essential for maintaining genomic integrity and ensuring accurate cell division. By monitoring critical processes and responding to abnormalities, checkpoints prevent the propagation of damaged DNA, thereby safeguarding against diseases such as cancer. Understanding these checkpoints is fundamental to developing therapeutic strategies targeting cell cycle dysregulation.
Q4. Describe the process of mitosis, outlining the key events that occur during each phase: prophase, metaphase, anaphase, and telophase.
Answer:
Mitosis Overview:
Mitosis is the process of nuclear division that ensures the equal distribution of duplicated chromosomes to two daughter cells. It consists of four main phases: prophase, metaphase, anaphase, and telophase, each characterized by distinct cellular events.
1. Prophase:
- Chromosome Condensation:
- Chromatin fibers condense into distinct, visible chromosomes.
- Each chromosome appears as two sister chromatids joined at the centromere.
- Nuclear Envelope Breakdown:
- The nuclear membrane disintegrates, allowing spindle fibers to interact with chromosomes.
- Mitotic Spindle Formation:
- Centrosomes (organelles that organize microtubules) migrate to opposite poles of the cell.
- Spindle fibers (microtubules) extend from the centrosomes, forming the mitotic spindle.
- Nucleolus Disappearance:
- The nucleolus fades as the nucleus prepares for division.
2. Metaphase:
- Chromosome Alignment:
- Chromosomes align at the cell’s equatorial plate (metaphase plate), an imaginary plane equidistant from the spindle poles.
- Spindle Fiber Attachment:
- Spindle fibers attach to the kinetochores, protein complexes located at the centromeres of each chromosome.
- Checkpoint Control:
- The spindle assembly checkpoint ensures that all chromosomes are correctly attached to spindle fibers before proceeding to anaphase.
3. Anaphase:
- Sister Chromatid Separation:
- Cohesin proteins holding sister chromatids together are cleaved, allowing chromatids to separate.
- Each chromatid is now considered an individual chromosome.
- Chromosome Movement:
- Spindle fibers shorten, pulling the separated chromosomes toward opposite poles of the cell.
- Poleward Movement:
- Chromosomes are rapidly moved to the spindle poles, ensuring each daughter cell will receive an identical set of chromosomes.
4. Telophase:
- Chromosome Decondensation:
- Chromosomes begin to decondense back into chromatin, becoming less visible.
- Nuclear Envelope Reformation:
- Nuclear membranes reassemble around each set of chromosomes, forming two distinct nuclei in the daughter cells.
- Spindle Disassembly:
- Mitotic spindle fibers disassemble and are recycled for future cell divisions.
- Nucleolus Reappearance:
- Nucleoli re-form within the newly established nuclei.
5. Cytokinesis (Concurrent with Telophase):
- Division of Cytoplasm:
- The cytoplasm divides, creating two separate daughter cells.
- In animal cells, a cleavage furrow forms, pinching the cell into two.
- In plant cells, a cell plate forms, developing into a new cell wall separating the daughter cells.
- Final Separation:
- Each daughter cell contains a complete set of chromosomes and its own nucleus, along with the necessary organelles to function independently.
Conclusion: Mitosis is a highly regulated process ensuring that each daughter cell receives an identical complement of chromosomes. The precise sequence of events in prophase, metaphase, anaphase, and telophase, coupled with cytokinesis, guarantees accurate genetic information transfer and maintains cellular and organismal integrity.
Q5. What are the roles of the anaphase-promoting complex/cyclosome (APC/C) in regulating mitosis and the cell cycle?
Answer:
Anaphase-Promoting Complex/Cyclosome (APC/C):
The APC/C is a crucial E3 ubiquitin ligase that regulates the progression of the cell cycle by targeting specific cell cycle proteins for degradation. It plays a pivotal role in controlling the transition from metaphase to anaphase and the exit from mitosis.
1. Structure and Composition:
- Multimeric Complex: The APC/C is a large protein complex composed of multiple subunits, which confer substrate specificity and regulatory control.
- Activation: Requires co-activator proteins such as Cdc20 and Cdh1, which are essential for substrate recognition.
2. Roles in Mitosis and Cell Cycle Regulation:
a. Triggering Anaphase: – Securin Degradation: – Function of Securin: Inhibits separase, an enzyme that cleaves cohesin complexes holding sister chromatids together. – APC/C Action: Targets securin for ubiquitination and subsequent degradation by the proteasome. – Outcome: Free separase becomes active, leading to the cleavage of cohesin and the separation of sister chromatids, initiating anaphase.
b. Cyclin Degradation: – Cyclin B Degradation: – Function of Cyclin B: Activates CDK1, promoting entry into mitosis. – APC/C Action: Ubiquitinates Cyclin B, targeting it for degradation. – Outcome: Inactivation of CDK1 leads to the exit from mitosis and the inactivation of mitotic processes.
c. Maintenance of Mitotic Exit: – Activation of Cdh1: – Function of Cdh1: Sustains APC/C activity during late mitosis and G₁ phase. – APC/C Action: Targets remaining mitotic cyclins and other proteins for degradation. – Outcome: Ensures complete exit from mitosis and prevents premature entry into the next cell cycle phase.
d. Regulation of the Cell Cycle Transition: – G₁/S Transition: – APC/C-Cdh1: Helps maintain low cyclin levels during G₁, preventing premature entry into S phase.
3. Regulation of APC/C Activity:
a. Spindle Assembly Checkpoint (SAC): – Function: Monitors chromosome attachment to the mitotic spindle. – APC/C Inhibition: When chromosomes are not properly attached, SAC proteins (e.g., Mad2) inhibit APC/C-Cdc20 activity, preventing anaphase onset. – Outcome: Ensures that anaphase does not proceed until all chromosomes are correctly aligned and attached, maintaining genomic stability.
b. Post-Mitosis Activation: – Switch from Cdc20 to Cdh1: After anaphase, Cdc20 is degraded or inactivated, and Cdh1 binds to APC/C to sustain its activity during mitotic exit and G₁ phase.
4. Consequences of APC/C Dysfunction:
a. Chromosome Segregation Errors: – Anaphase Delay or Failure: Impaired APC/C activity can prevent securin degradation, leading to sister chromatid cohesion and chromosome missegregation. – Aneuploidy: Results in daughter cells with abnormal chromosome numbers, contributing to cancer and developmental disorders.
b. Mitotic Catastrophe: – Uncontrolled Mitosis: Failure to degrade Cyclin B can cause prolonged mitosis, leading to cell death or uncontrolled cell proliferation.
c. Tumorigenesis: – Genomic Instability: APC/C dysfunction is associated with various cancers due to the accumulation of mitotic cyclins and improper chromosome segregation.
d. Developmental Defects: – Cell Cycle Dysregulation: Essential for proper cell division during development; defects can lead to developmental abnormalities.
Conclusion: The APC/C is a vital regulator of mitosis and the cell cycle, orchestrating the timely degradation of key proteins to ensure accurate chromosome segregation and cell cycle progression. Its precise control is essential for maintaining genomic integrity, and its dysfunction is implicated in numerous diseases, particularly cancer. Understanding APC/C function provides insights into cell cycle regulation and potential therapeutic targets for cell division-related disorders.
Q6. How do cell cycle checkpoints interact with cyclin-CDK complexes to control cell cycle progression?
Answer:
Interaction Between Cell Cycle Checkpoints and Cyclin-CDK Complexes:
Cell cycle checkpoints are critical regulatory mechanisms that monitor the integrity and completeness of cellular processes before allowing progression to subsequent cell cycle phases. Cyclin-CDK complexes are the primary drivers of cell cycle transitions, and their activity is tightly regulated by checkpoints to ensure accurate and orderly cell division.
1. Overview of Cyclin-CDK Complexes:
- Cyclins: Regulatory proteins whose levels fluctuate throughout the cell cycle.
- CDKs (Cyclin-Dependent Kinases): Kinases that, when bound to cyclins, phosphorylate target proteins to drive cell cycle progression.
- Activation: Cyclin binding and phosphorylation by CDK-activating kinases (CAKs) activate the cyclin-CDK complexes.
2. Cell Cycle Checkpoints:
a. G₁ Checkpoint: – Function: Assesses cell size, nutrient availability, and DNA integrity before entering S phase. – Cyclin-CDK Involvement: Cyclin D-CDK4/6 and Cyclin E-CDK2 drive G₁ to S transition. – Checkpoint Interaction: – p53 Activation: In response to DNA damage, p53 induces the expression of p21, a CDK inhibitor (CKI). – p21 Inhibition: Binds to Cyclin E-CDK2 and Cyclin D-CDK4/6 complexes, inhibiting their kinase activity. – Outcome: Halts cell cycle progression, allowing time for DNA repair or triggering apoptosis if damage is irreparable.
b. G₂ Checkpoint: – Function: Verifies that DNA replication in S phase has been completed successfully and that there is no DNA damage before entering mitosis. – Cyclin-CDK Involvement: Cyclin A-CDK2 and Cyclin B-CDK1 promote G₂ to M transition. – Checkpoint Interaction: – ATM/ATR Activation: Detect DNA damage or incomplete replication, activating checkpoint kinases Chk1 and Chk2. – Chk1/Chk2 Action: Phosphorylate and activate p53 or inhibit Cdc25 phosphatases. – Cdc25 Inhibition: Prevents activation of Cyclin B-CDK1 by maintaining it in an inactive, phosphorylated state. – Outcome: Delays mitotic entry, allowing DNA repair or halting the cycle if damage persists.
c. Spindle Assembly Checkpoint (Metaphase Checkpoint): – Function: Ensures all chromosomes are properly attached to the mitotic spindle before anaphase begins. – Cyclin-CDK Involvement: Cyclin B-CDK1 drives entry into mitosis. – Checkpoint Interaction: – Checkpoint Proteins (MAD2, BUBR1): Detect unattached kinetochores and inhibit the Anaphase-Promoting Complex/Cyclosome (APC/C) via Cdc20. – APC/C Inhibition: Prevents degradation of securin and Cyclin B, halting progression to anaphase. – Outcome: Ensures accurate chromosome segregation, preventing aneuploidy.
3. Cyclin-CDK Regulation by Checkpoints:
- Activation Control: Checkpoints can inhibit cyclin-CDK activity through CKIs or by preventing the activation of CDKs.
- Degradation of Cyclins: Checkpoints may promote the degradation of cyclins, reducing CDK activity and halting the cycle.
- Phosphorylation of CDKs: Checkpoints can modify CDKs post-translationally to alter their activity or interactions.
4. Integration of Checkpoints and Cyclin-CDK Activity:
- Feedback Loops: Active cyclin-CDK complexes can influence checkpoint activity, creating feedback mechanisms that stabilize cell cycle control.
- Cross-Talk: Signals from different checkpoints can converge on cyclin-CDK complexes, coordinating responses to various cellular conditions.
5. Consequences of Checkpoint and Cyclin-CDK Dysregulation:
- Uncontrolled Cell Proliferation: Failure of checkpoints to inhibit cyclin-CDK complexes can lead to unchecked cell division, contributing to cancer.
- Genomic Instability: Inadequate checkpoint control can result in the propagation of DNA damage, mutations, and chromosomal aberrations.
- Cell Cycle Arrest or Apoptosis: Overactive checkpoints can unnecessarily halt the cell cycle or induce cell death, impacting tissue regeneration and function.
Conclusion: Cell cycle checkpoints and cyclin-CDK complexes work in tandem to regulate cell cycle progression, ensuring that cells only proceed to the next phase when conditions are favorable and cellular integrity is maintained. This interplay is vital for preventing genomic instability and maintaining proper cell function, with dysregulation leading to diseases such as cancer. Understanding these regulatory mechanisms is fundamental for developing targeted therapies that can correct or exploit cell cycle abnormalities in disease contexts.
Q7. What is the role of the retinoblastoma protein (Rb) in regulating the G₁ checkpoint, and how does its phosphorylation status affect cell cycle progression?
Answer:
Retinoblastoma Protein (Rb) Overview:
The retinoblastoma protein (Rb) is a crucial tumor suppressor involved in regulating the G₁ checkpoint of the cell cycle. It plays a key role in controlling cell cycle progression from G₁ to S phase by modulating the activity of transcription factors that drive DNA replication.
1. Function of Rb:
Transcriptional Regulation:
- E2F Transcription Factors: Rb binds to E2F transcription factors, inhibiting their ability to activate genes required for S phase entry and DNA replication.
- Gene Repression: When bound to E2F, Rb represses the transcription of genes involved in DNA synthesis, such as cyclins, CDKs, and DNA polymerases.
Cell Cycle Control:
- Inhibition of Proliferation: By sequestering E2F, Rb prevents cells from prematurely entering S phase, thereby inhibiting uncontrolled cell proliferation.
- Differentiation and Apoptosis: Rb also plays roles in promoting cellular differentiation and apoptosis in response to oncogenic signals.
2. Regulation by Cyclin-CDK Complexes:
- Cyclin D-CDK4/6 Activation:
- Initiation: In response to mitogenic signals, cyclin D levels increase, binding to CDK4/6.
- Phosphorylation of Rb: The active cyclin D-CDK4/6 complex phosphorylates Rb on multiple serine and threonine residues.
- Cyclin E-CDK2 Activation:
- Further Phosphorylation: As the cell progresses through G₁, cyclin E levels rise, binding to CDK2.
- Additional Phosphorylation of Rb: Cyclin E-CDK2 further phosphorylates Rb, enhancing its inactivation.
3. Effects of Rb Phosphorylation Status:
Hypophosphorylated (Active) Rb:
- E2F Binding: Rb tightly binds E2F transcription factors, preventing them from activating S phase genes.
- Cell Cycle Arrest: Maintains the cell in G₁ phase, inhibiting progression to S phase.
Hyperphosphorylated (Inactive) Rb:
- E2F Release: Phosphorylation causes conformational changes in Rb, releasing E2F transcription factors.
- Gene Activation: Free E2F activates the transcription of genes necessary for DNA replication and S phase entry.
- Cell Cycle Progression: Allows the cell to proceed from G₁ to S phase, committing to DNA synthesis and division.
4. Regulation by Tumor Suppressors and Oncogenes:
- p53 Pathway:
- Response to DNA Damage: In response to DNA damage, p53 is activated and induces the expression of p21, a CDK inhibitor.
- p21 Inhibition: p21 binds to cyclin-CDK complexes, preventing Rb phosphorylation and maintaining Rb in its active, growth-suppressive state.
- Oncogenes:
- Overactivation of Cyclin-CDK: Oncogenes that drive overexpression of cyclins or CDKs can lead to excessive Rb phosphorylation, bypassing the G₁ checkpoint and promoting uncontrolled cell proliferation.
5. Clinical Implications:
- Retinoblastoma Disease:
- RB1 Gene Mutation: Loss or mutation of the RB1 gene, encoding Rb, leads to loss of cell cycle control.
- Tumor Formation: Unregulated E2F activity promotes excessive cell division, contributing to the development of retinoblastoma, a rare childhood eye cancer.
- Cancer Development:
- Rb Dysfunction: Alterations in Rb function are implicated in various cancers, where the loss of Rb-mediated growth suppression facilitates tumorigenesis.
Conclusion: The retinoblastoma protein (Rb) is a vital regulator of the G₁ checkpoint, controlling the transition to S phase by inhibiting E2F transcription factors. Its phosphorylation by cyclin-CDK complexes inactivates Rb, allowing cell cycle progression. Proper regulation of Rb is essential for maintaining cell cycle fidelity, and its dysfunction is a key event in the development of several cancers. Understanding Rb’s role provides insights into cell cycle control mechanisms and potential therapeutic targets for cancer treatment.
Q8. How does the spindle assembly checkpoint (SAC) function to prevent chromosomal missegregation during mitosis?
Answer:
Spindle Assembly Checkpoint (SAC) Overview:
The spindle assembly checkpoint (SAC) is a critical regulatory mechanism that ensures accurate chromosome segregation during mitosis. It monitors the attachment and alignment of chromosomes to the mitotic spindle, preventing the onset of anaphase until all chromosomes are properly positioned.
1. Function of SAC:
- Prevention of Premature Anaphase: SAC halts cell cycle progression from metaphase to anaphase if any chromosome is not correctly attached to spindle microtubules.
- Ensuring Biased Chromosome Segregation: Guarantees that each daughter cell receives an identical and complete set of chromosomes, maintaining genomic stability.
2. Key Components and Mechanism:
a. Detection of Unattached Kinetochores: – Kinetochores: Protein complexes assembled on the centromere of each chromosome, serving as attachment points for spindle microtubules. – Unattached Kinetochores: If a kinetochore lacks proper microtubule attachment, it generates a SAC signal.
b. Activation of the SAC Signal: – Checkpoint Proteins: Proteins such as Mad1, Mad2, Bub1, Bub3, and BubR1 accumulate at unattached kinetochores. – Mitotic Checkpoint Complex (MCC): Mad2 and BubR1 form a complex that inhibits the Anaphase-Promoting Complex/Cyclosome (APC/C) by sequestering its activator Cdc20.
c. Inhibition of APC/C: – APC/C Function: Normally targets securin and Cyclin B for degradation, allowing separase activation and Cyclin-CDK inactivation, leading to anaphase onset. – SAC Inhibition: By blocking APC/C-Cdc20 interaction, SAC prevents securin degradation, maintaining cohesin-mediated sister chromatid cohesion. – Outcome: Delays anaphase until all chromosomes are correctly attached and aligned.
d. Correction of Attachment Errors: – Aurora Kinases and Kinetochore Dynamics: SAC influences the activity of Aurora kinases, which regulate microtubule dynamics and kinetochore-microtubule attachments, facilitating error correction. – Microtubule Detachment and Reattachment: SAC allows time for improper attachments to be released and re-established correctly.
3. Silencing the SAC Signal:
- Biorientation Achievement: Once all kinetochores are properly attached to spindle microtubules from opposite poles (biorientation) and aligned at the metaphase plate, SAC proteins are released.
- APC/C Activation: With SAC inhibition lifted, APC/C can activate by binding Cdc20, leading to securin and Cyclin B degradation.
- Anaphase Initiation: Separase cleaves cohesin, allowing sister chromatids to separate and move to opposite poles, progressing the cell cycle into anaphase.
4. Consequences of SAC Failure:
- Chromosomal Missegregation: If SAC fails to detect unattached kinetochores, anaphase may proceed prematurely, resulting in daughter cells with abnormal chromosome numbers (aneuploidy).
- Genomic Instability: Aneuploidy and chromosomal rearrangements contribute to tumorigenesis and are hallmarks of many cancers.
- Cell Death: Severe missegregation can trigger cell death pathways, leading to apoptosis or necrosis.
5. Therapeutic Implications:
- Cancer Treatment: Many anti-cancer drugs target SAC components or spindle dynamics to induce mitotic arrest and promote cell death in rapidly dividing tumor cells.
- Drug Resistance: Alterations in SAC components can lead to resistance to chemotherapeutic agents that rely on SAC-mediated mitotic arrest for their efficacy.
Conclusion: The spindle assembly checkpoint (SAC) is essential for ensuring accurate chromosome segregation during mitosis by monitoring kinetochore-microtubule attachments. By preventing the transition to anaphase until all chromosomes are correctly attached and aligned, SAC maintains genomic stability and prevents aneuploidy. Dysregulation of SAC function is associated with cancer and other diseases, highlighting its importance in cell cycle control and as a target for therapeutic interventions.
Q9. Explain the process of cytokinesis in animal and plant cells, highlighting the differences between the two.
Answer:
Cytokinesis Overview:
Cytokinesis is the final step of the cell cycle, involving the division of the cytoplasm to produce two distinct daughter cells. Although the fundamental goal is the same in both animal and plant cells, the mechanisms differ due to structural differences in their cell walls and membrane systems.
1. Cytokinesis in Animal Cells:
a. Cleavage Furrow Formation: – Actin-Myosin Contractile Ring: A ring composed of actin filaments and myosin motor proteins assembles just beneath the plasma membrane at the cell’s equatorial region. – Contraction: Myosin motors slide along actin filaments, causing the contractile ring to tighten and pull the plasma membrane inward. – Furrow Deepening: The progressive contraction of the ring deepens the cleavage furrow, ultimately pinching the cell into two separate daughter cells.
b. Completion of Division: – Membrane Pinching: The furrow continues to constrict until the plasma membrane separates, completing cytokinesis. – Organelle Distribution: Organelles are equally partitioned between the two daughter cells, ensuring each cell has the necessary components for survival.
c. Role of the Central Spindle: – Mitotic Spindle Microtubules: The central spindle, composed of antiparallel microtubules between the separating chromosomes, helps position the contractile ring and cleavage furrow. – Rho GTPases: Proteins like RhoA regulate the assembly and contraction of the actin-myosin ring, coordinating cytokinesis.
2. Cytokinesis in Plant Cells:
a. Cell Plate Formation: – Phragmoplast Assembly: A structure made of microtubules, actin filaments, and vesicles forms in the central region of the cell between the segregating chromosomes. – Vesicle Transport: Golgi-derived vesicles carrying cell wall materials (cellulose, hemicellulose, pectin) accumulate at the center of the phragmoplast. – Fusion of Vesicles: Vesicles fuse to form the cell plate, a new membrane-bound structure that expands outward toward the existing cell walls.
b. Maturation of the Cell Plate: – Cell Wall Formation: Enzymes and structural proteins incorporated into the cell plate facilitate the synthesis of new cell wall materials, integrating the cell plate into the existing walls. – Expansion: The cell plate continues to grow until it fuses with the parental cell wall, effectively dividing the cell into two separate daughter cells.
c. Structural Considerations: – Rigid Cell Wall: Unlike animal cells, plant cells have a rigid cell wall that prevents the inward pinching mechanism of cytokinesis. Instead, they utilize the outward expansion of the cell plate to achieve division.
3. Key Differences Between Animal and Plant Cytokinesis:
Feature | Animal Cells | Plant Cells |
---|---|---|
Division Mechanism | Cleavage furrow formation via contractile ring | Cell plate formation via phragmoplast and vesicle fusion |
Structural Components | Actin-myosin contractile ring | Phragmoplast (microtubules and vesicles) |
Force Direction | Inward constriction of plasma membrane | Outward expansion of cell plate |
Cell Wall Consideration | No rigid cell wall; plasma membrane divides | Rigid cell wall; requires building new cell wall materials |
Regulatory Proteins | Rho GTPases (e.g., RhoA) | Similar regulatory proteins involved in vesicle trafficking and fusion |
Final Separation | Pinching leads to two daughter cells | Cell plate integrates into existing walls, forming two cells |
4. Functional Implications:
- Animal Cells:
- Flexibility: The cleavage furrow allows rapid and flexible cell division, accommodating the dynamic nature of animal tissues.
- Asymmetric Division: Facilitates asymmetric cell divisions crucial for differentiation and development.
- Plant Cells:
- Structural Integrity: The cell plate ensures that each daughter cell maintains a complete and robust cell wall, essential for plant structural integrity.
- Symmetric Division: Typically results in symmetric cell divisions, promoting uniformity in plant tissue organization.
Conclusion: Cytokinesis in animal and plant cells employs distinct mechanisms tailored to their structural differences. Animal cells utilize an inward-cleaving contractile ring to divide the plasma membrane, while plant cells build an outward-growing cell plate to integrate into their rigid cell walls. Understanding these processes highlights the diversity of cellular division strategies adapted to different organismal architectures and functional requirements.
Q10. What are the consequences of dysregulated cell cycle progression, and how do these contribute to the development of cancer?
Answer:
Consequences of Dysregulated Cell Cycle Progression:
Proper regulation of the cell cycle is essential for maintaining cellular and organismal homeostasis. Dysregulation can lead to uncontrolled cell proliferation, genomic instability, and evasion of normal growth constraints, all of which are hallmarks of cancer development.
1. Uncontrolled Cell Proliferation:
- Loss of Checkpoint Control: Failure of cell cycle checkpoints (e.g., G₁, G₂, metaphase) to halt progression in response to DNA damage or incomplete replication allows cells to divide despite abnormalities.
- Overactive Cyclin-CDK Complexes: Excessive activity of cyclin-CDK complexes drives continuous cell cycle progression without proper regulation.
- Example: Overexpression of Cyclin D or CDK4 can lead to unchecked progression through the G₁ phase, promoting rapid cell division.
2. Genomic Instability:
- Chromosomal Aberrations: Inaccurate DNA replication and segregation result in mutations, deletions, duplications, and translocations.
- Aneuploidy: Abnormal number of chromosomes in daughter cells disrupts gene dosage and cellular function.
- Telomere Dysfunction: Shortened telomeres can lead to chromosomal instability, while telomerase activation allows indefinite replication.
- Example: Mutations in genes responsible for DNA repair (e.g., BRCA1, BRCA2) increase the risk of genomic instability and cancer.
3. Evasion of Apoptosis:
- Inhibition of Programmed Cell Death: Cancer cells often develop mechanisms to evade apoptosis, allowing survival despite DNA damage or oncogenic stress.
- Overexpression of Anti-Apoptotic Proteins: Proteins like Bcl-2 inhibit apoptotic pathways.
- Example: Loss of p53 function prevents the induction of apoptosis in response to DNA damage, enabling survival of abnormal cells.
4. Loss of Contact Inhibition:
- Continued Growth in Dense Cultures: Normal cells exhibit contact inhibition, halting division upon cell-cell contact. Cancer cells lose this property, leading to unregulated growth.
- Formation of Multilayered Cell Structures: Results in disorganized tissue architecture.
- Example: Mutations in genes regulating cell adhesion (e.g., E-cadherin) disrupt contact inhibition and promote tumor growth.
5. Sustained Angiogenesis:
- Formation of New Blood Vessels: Tumors promote angiogenesis to supply nutrients and oxygen, supporting their growth and enabling metastasis.
- Upregulation of Angiogenic Factors: Proteins like VEGF are overexpressed in cancer cells.
- Example: Increased VEGF expression facilitates the development of blood vessels that nourish expanding tumors.
6. Metastasis:
- Invasion of Surrounding Tissues: Cancer cells acquire the ability to invade adjacent tissues and enter the bloodstream or lymphatic system.
- Colonization of Distant Organs: Enables the formation of secondary tumors.
- Example: Alterations in cell adhesion molecules and extracellular matrix-degrading enzymes (e.g., MMPs) facilitate metastasis.
7. Activation of Oncogenes and Inactivation of Tumor Suppressors:
- Oncogenes: Mutated or overexpressed genes (e.g., Ras, Myc) drive cell proliferation and survival.
- Tumor Suppressors: Loss or inactivation of genes (e.g., p53, RB) removes growth restraints and apoptotic signals.
- Example: Mutation of the Ras gene leads to constitutive activation of signaling pathways promoting cell growth and division.
8. Resistance to Growth Inhibitors:
- Insensitivity to Regulatory Signals: Cancer cells can bypass inhibitory signals that normally restrain cell cycle progression.
- Example: Loss of function in TGF-β signaling pathways removes inhibitory controls on cell proliferation.
9. Altered Metabolism:
- Warburg Effect: Cancer cells preferentially utilize glycolysis for energy production, even in the presence of oxygen, supporting rapid growth and biosynthesis.
- Metabolic Adaptations: Enable survival and proliferation under various conditions.
- Example: Increased glucose uptake and lactate production facilitate the energetic and biosynthetic demands of cancer cells.
10. Immune Evasion:
- Avoidance of Immune Surveillance: Cancer cells develop mechanisms to evade detection and destruction by the immune system.
- Example: Expression of immune checkpoint proteins (e.g., PD-L1) inhibits T cell activity against tumor cells.
Conclusion: Dysregulated cell cycle progression is a fundamental aspect of cancer development, leading to uncontrolled proliferation, genomic instability, evasion of apoptosis, and other malignant traits. Understanding the molecular mechanisms underlying cell cycle dysregulation provides insights into cancer pathogenesis and identifies potential targets for therapeutic intervention. Effective cancer treatments often aim to restore normal cell cycle control, induce apoptosis, inhibit angiogenesis, and prevent metastasis, addressing the multifaceted nature of dysregulated cell cycles in cancer cells.
Q11. How do tumor suppressor genes and oncogenes interact to regulate the cell cycle, and what happens when their functions are altered?
Answer:
Tumor Suppressor Genes and Oncogenes Overview:
Tumor suppressor genes and oncogenes are two categories of genes that play critical roles in regulating the cell cycle and maintaining cellular homeostasis. Their balanced interactions are essential for preventing uncontrolled cell proliferation and tumor formation.
1. Tumor Suppressor Genes:
Definition: Genes that encode proteins responsible for inhibiting cell division, repairing DNA, and inducing apoptosis. They act as brakes on the cell cycle.
Function:
- Cell Cycle Inhibition: Prevent progression to the next cell cycle phase when conditions are unfavorable.
- DNA Repair: Facilitate the correction of DNA damage, maintaining genomic integrity.
- Apoptosis Induction: Trigger programmed cell death in cells with irreparable damage.
Key Examples:
- TP53 (p53): Activates DNA repair proteins, induces cell cycle arrest via p21, and promotes apoptosis.
- RB1 (Retinoblastoma Protein): Inhibits E2F transcription factors, preventing G₁ to S phase transition.
- BRCA1/BRCA2: Involved in homologous recombination repair of double-strand breaks.
Alterations and Consequences:
- Loss of Function: Mutations, deletions, or epigenetic silencing of tumor suppressor genes reduce their inhibitory effects.
- Impact: Unregulated cell cycle progression, accumulation of DNA damage, and increased risk of cancer.
- Example: Mutation of TP53 is one of the most common alterations in human cancers, leading to impaired DNA damage response and apoptosis.
2. Oncogenes:
Definition: Genes that, when mutated or overexpressed, promote cell division, survival, and other processes that contribute to tumorigenesis. They act as gas pedals for the cell cycle.
Function:
- Cell Cycle Promotion: Enhance progression through cell cycle phases by activating cyclin-CDK complexes or downstream signaling pathways.
- Cell Survival and Growth: Support cellular proliferation and prevent apoptosis.
Key Examples:
- RAS: Encodes a GTPase involved in transmitting growth signals; mutations lead to constitutive activation.
- MYC: Transcription factor that drives expression of genes involved in cell growth and proliferation.
- HER2/neu (ERBB2): Receptor tyrosine kinase that, when overexpressed, promotes aggressive cell division.
Alterations and Consequences:
- Gain of Function: Mutations, gene amplification, or chromosomal translocations result in increased or unregulated activity.
- Impact: Enhanced cell proliferation, resistance to apoptosis, and increased potential for metastasis.
- Example: Amplification of HER2/neu in breast cancer leads to aggressive tumor growth and poor prognosis.
3. Interaction Between Tumor Suppressors and Oncogenes:
Balance of Signals: Tumor suppressor genes and oncogenes provide opposing signals that regulate cell cycle progression. A balance between these signals ensures controlled cell division.
Disruption of Balance: Alterations in either category can tip the balance towards uncontrolled proliferation and tumorigenesis.
- Loss of Tumor Suppressors + Activation of Oncogenes: Synergistically drive cancer progression by removing inhibitory controls and promoting proliferative signals.
- Example: Concurrent mutation of TP53 (loss of tumor suppressor) and activation of RAS (oncogene) significantly increases the likelihood of malignant transformation.
4. Mechanisms of Alteration:
a. Tumor Suppressor Genes: – Mutations: Point mutations, insertions, deletions that disrupt protein function. – Gene Deletions: Loss of entire gene copies, reducing protein levels. – Epigenetic Silencing: Methylation of promoter regions inhibits gene expression.
b. Oncogenes: – Point Mutations: Convert proto-oncogenes into constitutively active oncogenes. – Gene Amplification: Increased copies lead to overexpression of the oncogenic protein. – Chromosomal Translocations: Create fusion genes with enhanced or aberrant function.
5. Clinical Implications:
Cancer Diagnosis and Prognosis:
- Genetic Profiling: Identifying mutations in tumor suppressors and oncogenes aids in diagnosing specific cancer types and predicting outcomes.
Targeted Therapies:
- Oncogene Inhibition: Drugs like tyrosine kinase inhibitors target overactive oncogenes (e.g., HER2 inhibitors in breast cancer).
- Restoration of Tumor Suppressor Function: Although challenging, strategies aim to restore or mimic tumor suppressor activities.
Personalized Medicine:
- Tailored Treatments: Based on the specific genetic alterations in a patient’s tumor, therapies can be customized for maximum efficacy.
Conclusion: Tumor suppressor genes and oncogenes are fundamental regulators of the cell cycle, maintaining the balance between cell division and growth inhibition. Alterations in these genes disrupt this balance, leading to uncontrolled proliferation and cancer development. Understanding the interactions and regulatory mechanisms between tumor suppressors and oncogenes is essential for developing effective cancer diagnostics and targeted therapies, ultimately improving patient outcomes.
Q12. How do cell cycle inhibitors, such as p21 and p27, function to halt cell cycle progression, and what is their significance in cancer prevention?
Answer:
Cell Cycle Inhibitors Overview:
Cell cycle inhibitors, including p21 (Cip1/Waf1) and p27 (Kip1), are crucial regulators that halt cell cycle progression in response to various internal and external signals. They function by inhibiting cyclin-CDK complexes, thereby enforcing cell cycle checkpoints and preventing uncontrolled cell division.
1. Function of p21 and p27:
a. p21 (Cip1/Waf1): – Regulation by p53: – Response to DNA Damage: Activated p53, a tumor suppressor, induces the expression of p21 in response to DNA damage or stress signals. – Inhibition of Cyclin-CDK Complexes: – Binding: p21 binds to and inhibits Cyclin E-CDK2 and Cyclin A-CDK2 complexes, blocking progression from G₁ to S phase. – Effect on Rb Phosphorylation: Prevents Rb phosphorylation, maintaining Rb in its active state and inhibiting E2F-mediated transcription of S phase genes. – Role in Cell Cycle Arrest: – Temporary Halt: Allows cells time to repair DNA damage before resuming the cell cycle. – Apoptosis Induction: In cases of severe damage, p21 can contribute to apoptotic pathways by interacting with other regulatory proteins.
b. p27 (Kip1): – Regulation by Growth Signals: – Response to Growth Inhibition: p27 levels increase in response to anti-mitogenic signals, such as contact inhibition or differentiation cues. – Inhibition of Cyclin-CDK Complexes: – Binding: p27 inhibits Cyclin D-CDK4/6 and Cyclin E-CDK2 complexes, preventing G₁ to S phase transition. – Effect on Rb Phosphorylation: Maintains Rb in an active state, blocking E2F-mediated gene expression. – Role in Cell Cycle Control: – Differentiation: Promotes cell cycle exit during differentiation. – Senescence: Contributes to cellular senescence by maintaining cell cycle arrest.
2. Mechanism of Inhibition:
- Direct Binding: Both p21 and p27 directly bind to cyclin-CDK complexes, obstructing substrate access and inhibiting kinase activity.
- Stoichiometric Inhibition: High concentrations of p21/p27 relative to cyclin-CDK complexes effectively suppress their activity.
- Regulation by Proteolysis:
- Skp2-Mediated Degradation: The SCF^Skp2 ubiquitin ligase complex targets p27 for proteasomal degradation, allowing cell cycle progression. Regulation of Skp2 influences p27 levels and cell cycle control.
3. Significance in Cancer Prevention:
- Tumor Suppression:
- Enforcement of Checkpoints: By inhibiting cyclin-CDK activity, p21 and p27 enforce cell cycle checkpoints, preventing the propagation of damaged or abnormal cells.
- Prevention of Uncontrolled Proliferation: Halts cell division in response to DNA damage or oncogenic signals, reducing the risk of tumorigenesis.
- Genomic Integrity Maintenance:
- DNA Repair Facilitation: Cell cycle arrest allows time for DNA repair mechanisms to correct mutations and prevent genomic instability.
- Promotion of Apoptosis:
- Elimination of Damaged Cells: In cases where DNA damage is irreparable, p21 and p27 can aid in directing cells towards apoptosis, eliminating potentially cancerous cells.
- Inhibition of Metastasis:
- Cell Cycle Exit: By promoting cell cycle arrest, p21 and p27 reduce the proliferation rate of cancer cells, limiting tumor growth and metastasis.
- Clinical Implications:
- Prognostic Markers: Low levels of p21 and p27 are often associated with poor prognosis in various cancers, as their absence facilitates unchecked cell division.
- Therapeutic Targets: Strategies to restore or mimic p21/p27 function are being explored as potential cancer treatments, aiming to reinstate cell cycle control and inhibit tumor growth.
4. Examples of p21 and p27 in Cancer:
- p21 Deficiency:
- Increased Tumorigenesis: Mice lacking p21 exhibit higher rates of spontaneous and chemically-induced tumors.
- Human Cancers: Reduced p21 expression is observed in various cancers, including breast, colon, and lung, correlating with advanced disease stages.
- p27 Downregulation:
- Poor Prognosis: Low p27 levels are linked to aggressive tumor behavior and decreased survival rates in cancers like prostate, gastric, and ovarian.
- Mechanisms: Overexpression of Skp2, which targets p27 for degradation, is common in cancers and contributes to reduced p27 levels.
Conclusion: Cell cycle inhibitors p21 and p27 are essential for maintaining proper cell cycle control, enforcing checkpoints, and preventing the proliferation of damaged or abnormal cells. Their ability to inhibit cyclin-CDK complexes makes them vital tumor suppressors. Dysregulation or loss of p21 and p27 function is frequently associated with cancer development and progression, underscoring their significance in cancer prevention and as targets for therapeutic intervention.
Conclusion: These twelve thought-provoking questions and detailed answers cover essential aspects of the cell cycle, including its phases, regulation by cyclins and CDKs, role of checkpoints, mitosis and cytokinesis processes, key regulatory proteins like Rb and APC/C, the impact of dysregulation on cancer development, and the function of cell cycle inhibitors. They are designed to deepen understanding, promote critical thinking, and reinforce key concepts essential for mastering cell biology. Utilizing these questions as study aids can help learners grasp the complexities of cell cycle regulation and its implications in health and disease.
Cell Cycle and Division: Thought-Provoking Questions
1. What are the distinct phases of the cell cycle, and what key processes occur in each phase?
Answer:
The cell cycle is a highly regulated sequence of events that lead to cell division and duplication. It consists of two main stages: Interphase and Mitotic (M) Phase, each subdivided into specific phases with distinct cellular activities.
1. Interphase:
- G₁ Phase (Gap 1):
- Cell Growth: The cell increases in size and synthesizes proteins and organelles necessary for DNA replication.
- RNA and Protein Synthesis: Production of mRNA and proteins essential for DNA synthesis.
- Preparation for DNA Replication: Ensures the cell has adequate resources and readiness to duplicate its DNA.
- S Phase (Synthesis):
- DNA Replication: Each chromosome is duplicated, resulting in sister chromatids connected at the centromere.
- Centrosome Duplication: Ensures each daughter cell will have its own centrosome to organize the mitotic spindle.
- G₂ Phase (Gap 2):
- Further Cell Growth: Additional growth and protein synthesis to prepare for mitosis.
- Microtubule Formation: Synthesis of microtubules necessary for spindle formation.
- Final Preparations: Checks for DNA replication accuracy and completion.
2. Mitotic (M) Phase:
- Mitosis:
- Prophase: Chromatin condenses into visible chromosomes, the nuclear envelope breaks down, and the mitotic spindle begins to form.
- Metaphase: Chromosomes align at the cell’s equatorial plate, ensuring each sister chromatid is attached to spindle fibers from opposite poles.
- Anaphase: Sister chromatids are pulled apart toward opposite poles of the cell, ensuring each daughter cell receives an identical set of chromosomes.
- Telophase: Chromosomes decondense back into chromatin, nuclear envelopes reform around each set of chromosomes, and the mitotic spindle disassembles.
- Cytokinesis:
- Division of Cytoplasm: In animal cells, a cleavage furrow forms and pinches the cell into two daughter cells. In plant cells, a cell plate forms to separate the two new cells.
- Completion: Each daughter cell contains a complete nucleus and a share of the cytoplasm and organelles.
Conclusion: Understanding the cell cycle’s phases is fundamental to comprehending how cells grow, replicate their DNA, and divide. Each phase is meticulously regulated to ensure accurate and efficient cell division, maintaining genetic stability across generations.
2. How do cyclins and cyclin-dependent kinases (CDKs) regulate the progression of the cell cycle?
Answer:
Cyclins and Cyclin-Dependent Kinases (CDKs):
Cyclins and CDKs are pivotal regulators of the cell cycle, acting as molecular switches that ensure the cell progresses through each phase in an orderly and controlled manner.
1. Cyclins:
- Definition: Regulatory proteins whose levels fluctuate throughout the cell cycle.
- Function: Activate CDKs by binding to them.
- Types:
- Cyclin D: Active during the G₁ phase.
- Cyclin E: Active at the G₁/S transition.
- Cyclin A: Active during the S and G₂ phases.
- Cyclin B: Active during the G₂/M transition.
2. Cyclin-Dependent Kinases (CDKs):
- Definition: Serine/threonine kinases that, when bound to cyclins, phosphorylate target proteins to drive cell cycle progression.
- Function: Catalyze the phosphorylation of specific substrates, initiating key events in the cell cycle.
3. Regulation Mechanism:
- Activation:
- Cyclin Synthesis: Cyclin levels increase at specific cell cycle phases.
- Cyclin-CDK Binding: Cyclins bind to their corresponding CDKs, activating the kinase.
- Phosphorylation of Targets: Activated CDKs phosphorylate proteins that promote progression to the next phase.
- Inhibition:
- CDK Inhibitors (CKIs): Proteins like p21 and p27 bind to cyclin-CDK complexes, inhibiting their activity.
- Degradation of Cyclins: Proteasomes degrade cyclins after they have fulfilled their role, leading to inactivation of CDKs.
- Feedback Loops: Positive and negative feedback mechanisms ensure proper timing and order of cell cycle events.
4. Specific Examples:
- G₁ to S Transition:
- Cyclin D-CDK4/6: Phosphorylates the retinoblastoma protein (Rb), releasing E2F transcription factors.
- E2F Activation: Promotes expression of genes required for DNA replication.
- S Phase Progression:
- Cyclin A-CDK2: Initiates DNA replication by activating replication machinery.
- G₂ to M Transition:
- Cyclin B-CDK1: Triggers the onset of mitosis by phosphorylating proteins involved in chromosome condensation and spindle formation.
5. Importance of Cyclin-CDK Regulation:
- Ensures Orderly Progression: Prevents the cell from entering a new phase before completing the current one.
- Prevents Uncontrolled Division: Dysregulation can lead to unchecked cell proliferation, contributing to cancer development.
- Facilitates Checkpoint Responses: Integrates signals from cell cycle checkpoints to halt progression if errors are detected.
Conclusion: Cyclins and CDKs form a tightly regulated system that governs the cell cycle’s progression. By controlling the activation and inactivation of CDKs through cyclin binding and degradation, cells ensure that division occurs accurately and in response to internal and external cues. This regulation is essential for maintaining genomic integrity and preventing diseases such as cancer.
3. What are the key checkpoints in the cell cycle, and how do they ensure the fidelity of cell division?
Answer:
Key Checkpoints in the Cell Cycle:
Cell cycle checkpoints are surveillance mechanisms that monitor the integrity and completion of critical cellular processes before allowing progression to the next phase. They ensure that cells do not pass on damaged or incomplete genetic material, maintaining genomic stability.
1. G₁ Checkpoint (Restriction Point):
- Location: Between G₁ phase and S phase.
- Function: Determines whether the cell has sufficient size, nutrients, and energy to proceed with DNA replication.
- Key Factors Monitored:
- DNA Integrity: Detects DNA damage.
- External Signals: Growth factors and environmental conditions.
- Cell Size and Metabolic Status: Ensures adequate resources for division.
- Regulation:
- Retinoblastoma Protein (Rb): Phosphorylation by Cyclin D-CDK4/6 releases E2F transcription factors, promoting S phase entry.
- p53 Tumor Suppressor: Activated in response to DNA damage, inducing expression of p21 CKI, which inhibits Cyclin-CDK complexes, halting the cell cycle.
2. G₂ Checkpoint:
- Location: Between G₂ phase and M phase.
- Function: Ensures that DNA replication in S phase has been accurately completed without errors.
- Key Factors Monitored:
- DNA Integrity: Detects DNA damage or incomplete replication.
- Replication Completion: Confirms that all chromosomes are fully replicated.
- Regulation:
- ATM/ATR Kinases: Detect DNA damage and activate p53 or other effectors.
- Cyclin B-CDK1 Inhibition: CKIs like p21 inhibit Cyclin B-CDK1, preventing entry into mitosis until issues are resolved.
3. Spindle Assembly Checkpoint (Metaphase Checkpoint):
- Location: During metaphase of mitosis.
- Function: Ensures that all chromosomes are properly attached to the mitotic spindle before anaphase begins.
- Key Factors Monitored:
- Chromosome Alignment: All chromosomes must be aligned at the metaphase plate.
- Spindle Attachment: Kinetochores of chromosomes must be attached to spindle fibers from opposite poles.
- Regulation:
- MAD and BUB Proteins: Inhibit the anaphase-promoting complex/cyclosome (APC/C) until all chromosomes are correctly attached.
- APC/C Activation: Once all chromosomes are properly attached, APC/C triggers the separation of sister chromatids and progression to anaphase.
4. G₀ Phase:
- Definition: A resting state where cells exit the cell cycle.
- Function: Allows cells to perform specialized functions without dividing.
- Re-entry Conditions: Cells can re-enter the cell cycle from G₀ in response to specific signals.
Mechanisms Ensuring Fidelity:
- DNA Repair Pathways: Activated at checkpoints to fix detected DNA damage before progression.
- Cell Cycle Arrest: Temporarily halts the cell cycle to allow time for repairs.
- Apoptosis Induction: If damage is irreparable, checkpoints can trigger programmed cell death to prevent propagation of mutations.
Consequences of Checkpoint Failures:
- Genomic Instability: Leads to mutations, chromosomal aberrations, and aneuploidy.
- Cancer Development: Checkpoint failures can result in uncontrolled cell division and tumor formation.
- Cell Death or Senescence: Severe checkpoint activation can lead to apoptosis or permanent cell cycle exit.
Conclusion: Cell cycle checkpoints are essential for maintaining genomic integrity and ensuring accurate cell division. By monitoring critical processes and responding to abnormalities, checkpoints prevent the propagation of damaged DNA, thereby safeguarding against diseases such as cancer. Understanding these checkpoints is fundamental to developing therapeutic strategies targeting cell cycle dysregulation.
4. How do feedback loops, both positive and negative, regulate signal transduction pathways to ensure appropriate cellular responses? Provide examples of each type of feedback in cell signaling.
Answer:
Feedback Loops in Signal Transduction Pathways:
Feedback loops are regulatory mechanisms where the output of a process influences its own activity, either enhancing (positive feedback) or inhibiting (negative feedback) the process. In cell signaling, feedback loops are crucial for maintaining homeostasis, controlling the intensity and duration of signals, and ensuring appropriate cellular responses.
1. Negative Feedback:
- Definition: The output of a signaling pathway acts to inhibit its own production or activity, preventing overactivation and maintaining balance.
- Function: Maintains homeostasis by ensuring that signals do not become excessively strong or prolonged.
- Mechanism: Involves inhibitory proteins or pathways that counteract the initial signal.
- Example:
- cAMP Pathway Regulation:
- Scenario: Activation of GPCRs stimulates adenylyl cyclase to produce cAMP, which activates Protein Kinase A (PKA).
- Negative Feedback: PKA phosphorylates and activates phosphodiesterase (PDE), an enzyme that breaks down cAMP into AMP.
- Outcome: Reduces cAMP levels, thereby decreasing PKA activity and attenuating the signal.
- cAMP Pathway Regulation:
2. Positive Feedback:
- Definition: The output of a signaling pathway enhances its own production or activity, amplifying the signal.
- Function: Drives processes to completion, ensuring decisive and robust cellular responses.
- Mechanism: Involves activatory proteins or pathways that reinforce the initial signal.
- Example:
- Blood Clotting Cascade:
- Scenario: Injury to a blood vessel exposes collagen, activating platelets and initiating the clotting cascade.
- Positive Feedback: Thrombin generated in the cascade converts fibrinogen to fibrin and activates more platelets, which in turn produce more thrombin.
- Outcome: Rapid and extensive formation of a blood clot to prevent excessive bleeding.
- Blood Clotting Cascade:
3. Balancing Positive and Negative Feedback:
- Integration of Signals: Cells often utilize a combination of both feedback types to fine-tune responses. For instance, the initial positive feedback in the LH surge is eventually balanced by negative feedback mechanisms to prevent excessive hormone levels.
4. Examples of Feedback Mechanisms in Cell Signaling:
- Hormonal Regulation: Elevated thyroid hormone levels inhibit the release of TRH and TSH through negative feedback.
- Reproductive Processes: During ovulation, a surge in LH is driven by positive feedback from high estrogen levels, which is later balanced by negative feedback to regulate hormone levels.
Conclusion: Feedback loops are fundamental for regulating signal transduction pathways, ensuring that cellular responses are appropriate, controlled, and sustainable. Negative feedback maintains equilibrium and prevents overstimulation, while positive feedback facilitates rapid and decisive actions. The interplay of these feedback mechanisms allows cells to effectively manage complex signaling environments and maintain physiological balance.
5. How do scaffolding proteins contribute to the specificity and efficiency of signaling pathways, and what are the potential consequences of their dysfunction?
Answer:
Scaffolding Proteins in Signal Transduction:
Scaffolding proteins are multifunctional proteins that organize and assemble specific signaling complexes by physically linking multiple components of a signaling pathway. They play a crucial role in enhancing the specificity, efficiency, and regulation of signal transduction.
1. Contributions to Specificity and Efficiency:
Spatial Organization:
- Localization of Signaling Components: Scaffolding proteins bring together kinases, phosphatases, and other signaling molecules in close proximity, ensuring that interactions occur only among designated partners.
- Example: KSR (Kinase Suppressor of Ras) scaffolds components of the MAPK/ERK pathway, facilitating efficient signal transmission from Raf to MEK to ERK.
Signal Amplification:
- Enhanced Interaction Rates: By tethering signaling molecules together, scaffolding proteins increase the likelihood of productive interactions, thereby amplifying the signal.
- Example: Ste5 in yeast organizes the kinases involved in the mating pheromone response, promoting robust activation of the MAPK cascade.
Prevention of Crosstalk:
- Pathway Segregation: Scaffolding proteins help segregate different signaling pathways, minimizing unintended interactions and maintaining pathway fidelity.
- Example: Axin scaffolds components of the Wnt signaling pathway, preventing interference from other pathways.
Temporal Coordination:
- Sequential Activation: Scaffolding proteins can coordinate the timing of signaling events, ensuring that each step of the pathway is activated in the correct order.
- Example: In the JNK signaling pathway, scaffold proteins ensure the timely activation of kinases, leading to precise regulation of gene expression.
Integration of Multiple Signals:
- Converging Inputs: Some scaffolding proteins can integrate signals from multiple receptors or pathways, allowing cells to coordinate complex responses.
- Example: Scaffold proteins in immune cells integrate signals from different receptors to modulate activation and response to pathogens.
2. Potential Consequences of Scaffolding Protein Dysfunction:
Loss of Signal Specificity:
- Increased Crosstalk: Without proper scaffolding, signaling molecules from different pathways may interact inappropriately, leading to mixed or unintended signals.
- Example: Disruption of KSR-mediated MAPK pathway assembly can result in aberrant activation of ERK by unrelated kinases.
Reduced Signal Efficiency:
- Inefficient Signaling: Absence or malfunction of scaffolding proteins can lead to decreased interaction rates among signaling components, weakening the overall signal.
- Example: Loss of Ste5 scaffolding in yeast impairs the pheromone response, reducing the cell’s ability to respond to mating signals.
Altered Signal Dynamics:
- Timing Disruptions: Malfunctioning scaffolding proteins can disrupt the temporal coordination of signaling events, leading to delayed or inappropriate cellular responses.
- Example: Impaired scaffolding in the Wnt pathway can affect the timing of β-catenin stabilization, altering gene expression patterns.
Disease Association:
- Cancer: Overactive scaffolding proteins can lead to persistent signaling pathways that drive uncontrolled cell proliferation and survival.
- Neurodegenerative Diseases: Impaired scaffolding in neuronal signaling pathways can disrupt synaptic function and neuronal health.
- Example: Mutations in axin scaffolding proteins are associated with colorectal cancer due to dysregulated Wnt signaling.
Developmental Defects:
- Impaired Tissue Formation: Scaffolding protein malfunctions can disrupt developmental signaling pathways, leading to congenital abnormalities and impaired tissue differentiation.
- Example: Defects in scaffold proteins involved in Hedgehog signaling can result in developmental disorders like holoprosencephaly.
Impaired Immune Responses:
- Dysregulated Signaling: Scaffolding protein malfunctions can affect immune cell activation and responses, compromising the immune system’s ability to fight infections.
- Example: Defective scaffolding in T-cell receptor signaling can impair T-cell activation and immune function.
Conclusion: Scaffolding proteins are essential for the precise and efficient operation of signal transduction pathways. By organizing and coordinating signaling complexes, they ensure that cellular responses are specific, robust, and appropriately regulated. Malfunctions in scaffolding proteins can lead to a wide range of biological dysfunctions and diseases, underscoring their critical role in maintaining cellular and organismal health.
6. How do receptor desensitization mechanisms function in G-Protein Coupled Receptor (GPCR) signaling, and what are the physiological consequences of impaired desensitization?
Answer:
Receptor Desensitization in GPCR Signaling:
Receptor desensitization is a regulatory process that reduces the responsiveness of G-Protein Coupled Receptors (GPCRs) to continuous or repeated stimulation by ligands. This mechanism prevents overstimulation, maintains cellular homeostasis, and ensures that cells can respond appropriately to fluctuating signal strengths.
Mechanisms of Receptor Desensitization:
Phosphorylation by GPCR Kinases (GRKs):
- Role of GRKs: GRKs specifically phosphorylate activated GPCRs on serine and threonine residues within the receptor’s cytoplasmic domains.
- Effect of Phosphorylation: Decreases the receptor’s affinity for G-proteins, reducing its ability to activate downstream signaling pathways.
Binding of Arrestin Proteins:
- Function of Arrestins: Arrestins (e.g., β-arrestin) bind to phosphorylated GPCRs, blocking further G-protein coupling.
- Additional Roles of Arrestins:
- Receptor Internalization: Facilitate the internalization of GPCRs through clathrin-coated pits, removing them from the cell surface and sequestering them for either recycling or degradation.
- β-Arrestin-Mediated Signaling: Arrestins can initiate alternative signaling pathways independent of G-proteins, contributing to diverse cellular responses.
Receptor Internalization and Downregulation:
- Endocytosis: Activated and phosphorylated GPCRs are internalized into the cell through endocytosis.
- Recycling vs. Degradation:
- Recycling: Receptors are returned to the cell surface for potential reuse.
- Degradation: Receptors are targeted for lysosomal degradation, leading to a decrease in receptor numbers and prolonged desensitization.
Regulation by Ubiquitination:
- Ubiquitin Ligases: Certain ubiquitin ligases tag desensitized receptors for degradation.
- Impact: Enhances receptor downregulation and prevents reactivation without new receptor synthesis.
Physiological Consequences of Impaired Desensitization:
Chronic Overstimulation:
- Persistent Signaling: Impaired desensitization leads to prolonged activation of GPCRs, causing continuous downstream signaling.
- Example: Chronic exposure to high levels of β-adrenergic agonists can result in excessive heart rate and contractility, potentially leading to cardiac hypertrophy.
Receptor Downregulation Defects:
- Receptor Overexpression: Failure to internalize and degrade desensitized receptors can result in receptor overexpression, enhancing sensitivity to ligands.
- Example: Overactive GPCR signaling is implicated in certain cancers, where receptors like β2-adrenergic receptors may remain active, promoting tumor growth and survival.
Tolerance Development:
- Pharmacological Tolerance: Impaired desensitization can prevent the development of tolerance to drugs targeting GPCRs, requiring higher doses for therapeutic effects.
- Example: In asthma treatment, inadequate desensitization of β2-adrenergic receptors can lead to reduced drug efficacy over time.
Immune System Dysregulation:
- Excessive Immune Responses: GPCR desensitization plays a role in regulating immune cell responses. Impaired desensitization can result in hyperactive immune cells, contributing to autoimmune diseases.
- Example: Overactive chemokine receptors on T cells can lead to excessive migration and inflammation in autoimmune conditions.
Neurological Disorders:
- Imbalanced Neurotransmission: GPCRs are critical in neurotransmitter signaling. Impaired desensitization can disrupt synaptic plasticity, affecting learning, memory, and mood regulation.
- Example: Dysregulated dopamine receptor desensitization is associated with psychiatric disorders like schizophrenia and addiction.
Cardiovascular Diseases:
- Hypertension and Heart Failure: Impaired desensitization of GPCRs involved in vascular tone regulation can contribute to hypertension and heart failure due to uncontrolled vasoconstriction and cardiac stress.
- Example: Persistent activation of angiotensin II receptors can lead to vasoconstriction, increased blood pressure, and adverse cardiac remodeling.
Conclusion: Receptor desensitization is a vital regulatory mechanism in GPCR signaling that ensures cells respond appropriately to varying levels of stimuli. Impaired desensitization disrupts this balance, leading to excessive or prolonged signaling that can contribute to a range of physiological and pathological conditions, including cardiovascular diseases, cancer, immune disorders, and neurological issues. Understanding desensitization mechanisms is essential for developing therapeutic strategies that target GPCRs effectively while minimizing adverse effects.
7. How do cells integrate multiple signaling pathways to produce a coordinated response, and what factors influence the outcome of such integration?
Answer:
Integration of Multiple Signaling Pathways:
Cells often receive and process multiple signals simultaneously, necessitating the integration of diverse signaling pathways to generate coherent and appropriate responses. This integration allows cells to respond dynamically to complex and changing environments, ensuring precise regulation of cellular functions.
Mechanisms of Signal Integration:
Convergence Points:
- Shared Downstream Effectors: Different signaling pathways may converge on common downstream molecules, enabling coordinated regulation.
- Example: Both the MAPK/ERK and PI3K/Akt pathways can influence gene expression through the activation of transcription factors like CREB.
Divergence Points:
- Branching Pathways: A single signaling molecule can activate multiple downstream pathways, leading to diverse cellular outcomes.
- Example: Activation of RTKs can simultaneously trigger the MAPK/ERK pathway for cell proliferation and the PI3K/Akt pathway for cell survival.
Cross-Talk Between Pathways:
- Direct Interactions: Components of one pathway can directly interact with components of another, modulating each other’s activity.
- Indirect Interactions: Pathways can influence each other through intermediary proteins or feedback loops.
- Example: The Wnt signaling pathway can interact with the PI3K/Akt pathway to regulate cell proliferation and survival.
Scaffold Proteins:
- Organizing Signaling Complexes: Scaffold proteins bring together multiple signaling molecules, enhancing specificity and efficiency.
- Example: KSR (Kinase Suppressor of Ras) scaffolds components of the MAPK/ERK pathway, ensuring efficient signal transmission and preventing crosstalk with unrelated pathways.
Temporal Dynamics:
- Sequential Activation: Signaling pathways can be activated in a specific temporal order, allowing one pathway to influence the activation of another.
- Example: Initial activation of a kinase cascade can prime cells to respond to subsequent signals by modifying transcription factors.
Spatial Localization:
- Compartmentalization: Signaling components localized to specific cellular regions (e.g., lipid rafts, endosomes) can selectively interact with certain pathways.
- Example: Signals initiated at the plasma membrane may differ from those initiated from endosomal compartments, leading to distinct outcomes.
Factors Influencing Signal Integration Outcomes:
Signal Strength and Duration:
- Intensity: The strength of each signal can determine the extent of pathway activation and the resultant cellular response.
- Duration: Prolonged versus transient signals can lead to different outcomes, such as sustained gene expression versus temporary metabolic changes.
Receptor Density and Distribution:
- Availability: The number and distribution of receptors on the cell surface influence the cell’s responsiveness to different signals.
- Example: High density of growth factor receptors may enhance sensitivity to mitogenic signals, promoting cell proliferation.
Scaffold and Adaptor Proteins:
- Organization of Signaling Complexes: Scaffold proteins facilitate specific interactions, ensuring that only relevant pathways are activated.
- Example: Axin scaffolds components of the Wnt signaling pathway, preventing interference from other pathways.
Feedback Mechanisms:
- Regulation of Pathway Activity: Positive and negative feedback loops can modulate the activity and integration of signaling pathways.
- Example: Negative feedback in the MAPK pathway can limit signal duration, allowing other pathways to influence the final response.
Post-Translational Modifications:
- Protein Modifications: Phosphorylation, ubiquitination, and other modifications can alter the activity, localization, or interactions of signaling proteins, affecting pathway integration.
- Example: Phosphorylation of a transcription factor by one pathway can influence its ability to be activated by another pathway.
Cellular Context and State:
- Differentiation Status: Stem cells versus differentiated cells may respond differently to the same signals due to variations in signaling machinery.
- Metabolic State: Nutrient availability and energy status can influence how signals are interpreted and integrated.
Competition for Signaling Components:
- Resource Allocation: Multiple pathways may compete for the same signaling molecules, affecting the overall outcome based on pathway priority and availability.
- Example: Competing activation of Raf by different upstream signals can influence the strength and specificity of the MAPK response.
Examples of Signal Integration:
Cell Cycle Regulation:
- Growth Factor and DNA Damage Signals: Integration of mitogenic signals (e.g., via RTKs) promoting cell cycle progression and stress signals (e.g., via p53) inducing cell cycle arrest or apoptosis.
- Outcome: Decision to proceed with division, repair DNA, or undergo apoptosis based on the balance of signals.
Immune Cell Activation:
- Antigen Recognition and Co-Stimulation: T cells integrate signals from the T cell receptor (antigen recognition) and co-stimulatory receptors (e.g., CD28) to fully activate immune responses.
- Outcome: Activation of T cells leads to proliferation, cytokine production, and differentiation into effector cells.
Neuronal Plasticity:
- Synaptic Activity and Growth Factors: Neurons integrate electrical signals from synaptic activity with growth factor signals to regulate synaptic strength and dendritic spine formation.
- Outcome: Modulation of synaptic connections underlying learning and memory.
Conclusion: Cells employ intricate mechanisms to integrate multiple signaling pathways, ensuring that responses are precise, coordinated, and appropriate to the cellular context. Factors such as signal strength, temporal dynamics, spatial localization, and the presence of scaffolding proteins play pivotal roles in determining the outcome of signal integration. Understanding how cells integrate signals is fundamental to elucidating complex biological processes and addressing dysregulated signaling in diseases.
8. How does the spindle assembly checkpoint (SAC) function to prevent chromosomal missegregation during mitosis?
Answer:
Spindle Assembly Checkpoint (SAC) Overview:
The spindle assembly checkpoint (SAC) is a critical regulatory mechanism that ensures accurate chromosome segregation during mitosis. It monitors the attachment and alignment of chromosomes to the mitotic spindle, preventing the onset of anaphase until all chromosomes are correctly positioned.
1. Function of SAC:
- Prevention of Premature Anaphase: SAC halts cell cycle progression from metaphase to anaphase if any chromosome is not properly attached to spindle microtubules.
- Ensuring Biased Chromosome Segregation: Guarantees that each daughter cell receives an identical and complete set of chromosomes, maintaining genomic stability.
2. Key Components and Mechanism:
- Kinetochores: Protein complexes assembled on the centromere of each chromosome, serving as attachment points for spindle microtubules.
- Checkpoint Proteins: Proteins such as Mad1, Mad2, Bub1, Bub3, and BubR1 accumulate at unattached kinetochores.
- Mitotic Checkpoint Complex (MCC): Mad2 and BubR1 form a complex that inhibits the Anaphase-Promoting Complex/Cyclosome (APC/C) by sequestering its activator Cdc20.
3. Mechanism of SAC Function:
- Detection of Unattached Kinetochores:
- Signal Generation: If a kinetochore lacks proper microtubule attachment, SAC proteins at the kinetochore activate and begin assembling the MCC.
- Inhibition of APC/C:
- APC/C Function: Normally targets securin and Cyclin B for degradation, allowing separase activation and Cyclin-CDK inactivation, leading to anaphase onset.
- SAC Inhibition: MCC binds to APC/C-Cdc20, preventing its activation.
- Outcome: Halts progression to anaphase until all chromosomes are correctly attached and aligned.
- Correction of Attachment Errors:
- Aurora Kinases and Kinetochore Dynamics: SAC influences the activity of Aurora kinases, which regulate microtubule dynamics and kinetochore-microtubule attachments, facilitating error correction.
- Microtubule Detachment and Reattachment: Allows time for improper attachments to be released and re-established correctly.
4. Silencing the SAC Signal:
- Biorientation Achievement: Once all kinetochores are properly attached to spindle microtubules from opposite poles (biorientation) and aligned at the metaphase plate, SAC proteins are released.
- APC/C Activation: With SAC inhibition lifted, APC/C can activate by binding Cdc20, leading to securin and Cyclin B degradation.
- Anaphase Initiation: Separase cleaves cohesin, allowing sister chromatids to separate and move to opposite poles, progressing the cell cycle into anaphase.
5. Consequences of SAC Failure:
- Chromosomal Missegregation: If SAC fails to detect unattached kinetochores, anaphase may proceed prematurely, resulting in daughter cells with abnormal chromosome numbers (aneuploidy).
- Genomic Instability: Aneuploidy and chromosomal rearrangements contribute to tumorigenesis and are hallmarks of many cancers.
- Cell Death: Severe missegregation can trigger cell death pathways, leading to apoptosis or necrosis.
6. Therapeutic Implications:
- Cancer Treatment: Many anti-cancer drugs target SAC components or spindle dynamics to induce mitotic arrest and promote cell death in rapidly dividing tumor cells.
- Drug Resistance: Alterations in SAC components can lead to resistance to chemotherapeutic agents that rely on SAC-mediated mitotic arrest for their efficacy.
Conclusion:
The spindle assembly checkpoint (SAC) is essential for ensuring accurate chromosome segregation during mitosis by monitoring kinetochore-microtubule attachments. By preventing the transition to anaphase until all chromosomes are correctly attached and aligned, SAC maintains genomic stability and prevents aneuploidy. Dysregulation of SAC function is associated with cancer and other diseases, highlighting its importance in cell cycle control and as a target for therapeutic interventions.
9. Explain the process of cytokinesis in animal and plant cells, highlighting the differences between the two.
Answer:
Cytokinesis Overview:
Cytokinesis is the final step of the cell cycle, involving the division of the cytoplasm to produce two distinct daughter cells. Although the fundamental goal is the same in both animal and plant cells, the mechanisms differ due to structural differences in their cell walls and membrane systems.
1. Cytokinesis in Animal Cells:
- Cleavage Furrow Formation:
- Actin-Myosin Contractile Ring: A ring composed of actin filaments and myosin motor proteins assembles just beneath the plasma membrane at the cell’s equatorial region.
- Contraction: Myosin motors slide along actin filaments, causing the contractile ring to tighten and pull the plasma membrane inward.
- Furrow Deepening: The progressive contraction of the ring deepens the cleavage furrow, ultimately pinching the cell into two separate daughter cells.
- Completion of Division:
- Membrane Pinching: The furrow continues to constrict until the plasma membrane separates, completing cytokinesis.
- Organelle Distribution: Organelles are equally partitioned between the two daughter cells, ensuring each cell has the necessary components for survival.
- Role of the Central Spindle:
- Mitotic Spindle Microtubules: The central spindle, composed of antiparallel microtubules between the separating chromosomes, helps position the contractile ring and cleavage furrow.
- Rho GTPases: Proteins like RhoA regulate the assembly and contraction of the actin-myosin ring, coordinating cytokinesis.
2. Cytokinesis in Plant Cells:
- Cell Plate Formation:
- Phragmoplast Assembly: A structure made of microtubules, actin filaments, and vesicles forms in the central region of the cell between the segregating chromosomes.
- Vesicle Transport: Golgi-derived vesicles carrying cell wall materials (cellulose, hemicellulose, pectin) accumulate at the center of the phragmoplast.
- Fusion of Vesicles: Vesicles fuse to form the cell plate, a new membrane-bound structure that expands outward toward the existing cell walls.
- Maturation of the Cell Plate:
- Cell Wall Formation: Enzymes and structural proteins incorporated into the cell plate facilitate the synthesis of new cell wall materials, integrating the cell plate into the existing walls.
- Expansion: The cell plate continues to grow until it fuses with the parental cell wall, effectively dividing the cell into two separate daughter cells.
- Structural Considerations:
- Rigid Cell Wall: Unlike animal cells, plant cells have a rigid cell wall that prevents the inward pinching mechanism of cytokinesis. Instead, they utilize the outward expansion of the cell plate to achieve division.
3. Key Differences Between Animal and Plant Cytokinesis:
Feature | Animal Cells | Plant Cells |
---|---|---|
Division Mechanism | Cleavage furrow formation via contractile ring | Cell plate formation via phragmoplast and vesicle fusion |
Structural Components | Actin-myosin contractile ring | Phragmoplast (microtubules and vesicles) |
Force Direction | Inward constriction of plasma membrane | Outward expansion of cell plate |
Cell Wall Consideration | No rigid cell wall; plasma membrane divides | Rigid cell wall; requires building new cell wall materials |
Regulatory Proteins | Rho GTPases (e.g., RhoA) | Similar regulatory proteins involved in vesicle trafficking and fusion |
Final Separation | Pinching leads to two daughter cells | Cell plate integrates into existing walls, forming two cells |
4. Functional Implications:
- Animal Cells:
- Flexibility: The cleavage furrow allows rapid and flexible cell division, accommodating the dynamic nature of animal tissues.
- Asymmetric Division: Facilitates asymmetric cell divisions crucial for differentiation and development.
- Plant Cells:
- Structural Integrity: The cell plate ensures that each daughter cell maintains a complete and robust cell wall, essential for plant structural integrity.
- Symmetric Division: Typically results in symmetric cell divisions, promoting uniformity in plant tissue organization.
Conclusion:
Cytokinesis in animal and plant cells employs distinct mechanisms tailored to their structural differences. Animal cells utilize an inward-cleaving contractile ring to divide the plasma membrane, while plant cells build an outward-growing cell plate to integrate into their rigid cell walls. Understanding these processes highlights the diversity of cellular division strategies adapted to different organismal architectures and functional requirements.
10. What are the consequences of dysregulated cell cycle progression, and how do these contribute to the development of cancer?
Answer:
Consequences of Dysregulated Cell Cycle Progression:
Proper regulation of the cell cycle is essential for maintaining cellular and organismal homeostasis. Dysregulation can lead to uncontrolled cell proliferation, genomic instability, and evasion of normal growth constraints, all of which are hallmarks of cancer development.
1. Uncontrolled Cell Proliferation:
- Loss of Checkpoint Control: Failure of cell cycle checkpoints (e.g., G₁, G₂, metaphase) to halt progression in response to DNA damage or incomplete replication allows cells to divide despite abnormalities.
- Overactive Cyclin-CDK Complexes: Excessive activity of cyclin-CDK complexes drives continuous cell cycle progression without proper regulation.
- Example: Overexpression of Cyclin D or CDK4 can lead to unchecked progression through the G₁ phase, promoting rapid cell division.
2. Genomic Instability:
- Chromosomal Aberrations: Inaccurate DNA replication and segregation result in mutations, deletions, duplications, and translocations.
- Aneuploidy: Abnormal number of chromosomes in daughter cells disrupts gene dosage and cellular function.
- Telomere Dysfunction: Shortened telomeres can lead to chromosomal instability, while telomerase activation allows indefinite replication.
- Example: Mutations in genes responsible for DNA repair (e.g., BRCA1, BRCA2) increase the risk of genomic instability and cancer.
3. Evasion of Apoptosis:
- Inhibition of Programmed Cell Death: Cancer cells often develop mechanisms to evade apoptosis, allowing survival despite DNA damage or oncogenic stress.
- Overexpression of Anti-Apoptotic Proteins: Proteins like Bcl-2 inhibit apoptotic pathways.
- Example: Loss of p53 function prevents the induction of apoptosis in response to DNA damage, enabling survival of abnormal cells.
4. Loss of Contact Inhibition:
- Continued Growth in Dense Cultures: Normal cells exhibit contact inhibition, halting division upon cell-cell contact. Cancer cells lose this property, leading to unregulated growth.
- Formation of Multilayered Cell Structures: Results in disorganized tissue architecture.
- Example: Mutations in genes regulating cell adhesion (e.g., E-cadherin) disrupt contact inhibition and promote tumor growth.
5. Sustained Angiogenesis:
- Formation of New Blood Vessels: Tumors promote angiogenesis to supply nutrients and oxygen, supporting their growth and enabling metastasis.
- Upregulation of Angiogenic Factors: Proteins like VEGF are overexpressed in cancer cells.
- Example: Increased VEGF expression facilitates the development of blood vessels that nourish expanding tumors.
6. Metastasis:
- Invasion of Surrounding Tissues: Cancer cells acquire the ability to invade adjacent tissues and enter the bloodstream or lymphatic system.
- Colonization of Distant Organs: Enables the formation of secondary tumors.
- Example: Alterations in cell adhesion molecules and extracellular matrix-degrading enzymes (e.g., MMPs) facilitate metastasis.
7. Activation of Oncogenes and Inactivation of Tumor Suppressors:
- Oncogenes: Mutated or overexpressed genes (e.g., Ras, Myc) drive cell proliferation and survival.
- Tumor Suppressors: Loss or inactivation of genes (e.g., p53, RB) removes growth restraints and apoptotic signals.
- Example: Mutation of the Ras gene leads to constitutive activation of signaling pathways promoting cell growth and division.
8. Resistance to Growth Inhibitors:
- Insensitivity to Regulatory Signals: Cancer cells can bypass inhibitory signals that normally restrain cell cycle progression.
- Example: Loss of function in TGF-β signaling pathways removes inhibitory controls on cell proliferation.
9. Altered Metabolism:
- Warburg Effect: Cancer cells preferentially utilize glycolysis for energy production, even in the presence of oxygen, supporting rapid growth and biosynthesis.
- Metabolic Adaptations: Enable survival and proliferation under various conditions.
- Example: Increased glucose uptake and lactate production facilitate the energetic and biosynthetic demands of cancer cells.
10. Immune Evasion:
- Avoidance of Immune Surveillance: Cancer cells develop mechanisms to evade detection and destruction by the immune system.
- Example: Expression of immune checkpoint proteins (e.g., PD-L1) inhibits T cell activity against tumor cells.
Conclusion:
Dysregulated cell cycle progression is a fundamental aspect of cancer development, leading to uncontrolled proliferation, genomic instability, evasion of apoptosis, and other malignant traits. Understanding the molecular mechanisms underlying cell cycle dysregulation provides insights into cancer pathogenesis and identifies potential targets for therapeutic intervention. Effective cancer treatments often aim to restore normal cell cycle control, induce apoptosis, inhibit angiogenesis, and prevent metastasis, addressing the multifaceted nature of dysregulated cell cycles in cancer cells.
10. How do cell cycle checkpoints interact with cyclin-CDK complexes to control cell cycle progression?
Answer:
Interaction Between Cell Cycle Checkpoints and Cyclin-CDK Complexes:
Cell cycle checkpoints are critical regulatory mechanisms that monitor the integrity and completion of essential cellular processes before allowing progression to subsequent phases. Cyclin-CDK complexes are the primary drivers of cell cycle transitions, and their activity is tightly regulated by checkpoints to ensure accurate and orderly cell division.
1. Overview of Cyclin-CDK Complexes:
- Cyclins: Regulatory proteins whose levels fluctuate throughout the cell cycle.
- CDKs (Cyclin-Dependent Kinases): Kinases that, when bound to cyclins, phosphorylate target proteins to drive cell cycle progression.
- Activation: Cyclin binding and phosphorylation by CDK-activating kinases (CAKs) activate the cyclin-CDK complexes.
2. Cell Cycle Checkpoints:
a. G₁ Checkpoint: – Function: Assesses cell size, nutrient availability, and DNA integrity before entering S phase. – Cyclin-CDK Involvement: Cyclin D-CDK4/6 and Cyclin E-CDK2 drive G₁ to S transition. – Checkpoint Interaction: – p53 Activation: In response to DNA damage, p53 induces the expression of p21, a CDK inhibitor (CKI). – p21 Inhibition: Binds to Cyclin E-CDK2 and Cyclin D-CDK4/6 complexes, inhibiting their kinase activity. – Outcome: Halts cell cycle progression, allowing time for DNA repair or triggering apoptosis if damage is irreparable.
b. G₂ Checkpoint: – Function: Verifies that DNA replication in S phase has been completed successfully and that there is no DNA damage before entering mitosis. – Cyclin-CDK Involvement: Cyclin A-CDK2 and Cyclin B-CDK1 promote G₂ to M transition. – Checkpoint Interaction: – ATM/ATR Activation: Detect DNA damage and activate checkpoint kinases Chk1 and Chk2. – Chk1/Chk2 Action: Phosphorylate and activate p53 or inhibit Cdc25 phosphatases. – Cdc25 Inhibition: Prevents activation of Cyclin B-CDK1 by maintaining it in an inactive, phosphorylated state. – Outcome: Delays mitotic entry, allowing DNA repair or halting the cycle if damage persists.
c. Spindle Assembly Checkpoint (Metaphase Checkpoint): – Function: Ensures that all chromosomes are properly attached to the mitotic spindle before anaphase begins. – Cyclin-CDK Involvement: Cyclin B-CDK1 drives entry into mitosis. – Checkpoint Interaction: – Checkpoint Proteins (MAD2, BUBR1): Detect unattached kinetochores and inhibit the Anaphase-Promoting Complex/Cyclosome (APC/C) via Cdc20. – APC/C Inhibition: Prevents degradation of securin and Cyclin B, halting progression to anaphase. – Outcome: Ensures accurate chromosome segregation, preventing aneuploidy.
3. Cyclin-CDK Regulation by Checkpoints:
- Activation Control: Checkpoints can inhibit cyclin-CDK activity through CKIs or by preventing the activation of CDKs.
- Degradation of Cyclins: Checkpoints may promote the degradation of cyclins, reducing CDK activity and halting the cycle.
- Phosphorylation of CDKs: Checkpoints can modify CDKs post-translationally to alter their activity or interactions.
4. Integration of Checkpoints and Cyclin-CDK Activity:
- Feedback Loops: Active cyclin-CDK complexes can influence checkpoint activity, creating feedback mechanisms that stabilize cell cycle control.
- Cross-Talk: Signals from different checkpoints can converge on cyclin-CDK complexes, coordinating responses to various cellular conditions.
5. Consequences of Checkpoint and Cyclin-CDK Dysregulation:
- Uncontrolled Cell Proliferation: Failure of checkpoints to inhibit cyclin-CDK complexes can lead to unchecked cell division, contributing to cancer.
- Genomic Instability: Inadequate checkpoint control can result in the propagation of DNA damage, mutations, and chromosomal aberrations.
- Cell Cycle Arrest or Apoptosis: Overactive checkpoints can unnecessarily halt the cell cycle or induce cell death, impacting tissue regeneration and function.
Conclusion:
Cell cycle checkpoints and cyclin-CDK complexes work in tandem to regulate cell cycle progression, ensuring that cells only proceed to the next phase when conditions are favorable and cellular integrity is maintained. This interplay is vital for preventing genomic instability and maintaining proper cell function, with dysregulation leading to diseases such as cancer. Understanding these regulatory mechanisms is fundamental for developing targeted therapies that can correct or exploit cell cycle abnormalities in disease contexts.
10. How do lysosomes contribute to cellular waste management, and what are the consequences of lysosomal dysfunction in human health?
Answer:
Lysosomes in Cellular Waste Management:
Lysosomes are membrane-bound organelles found in eukaryotic cells that function as the cell’s waste disposal system. They contain a variety of hydrolytic enzymes capable of breaking down all types of biological polymers, including proteins, nucleic acids, carbohydrates, and lipids. Lysosomes play a crucial role in degrading and recycling cellular waste, damaged organelles, and engulfed pathogens.
Functions of Lysosomes:
Intracellular Digestion:
- Autophagy: Lysosomes digest and recycle damaged or obsolete organelles and macromolecules within the cell. Autophagosomes engulf the targeted components and fuse with lysosomes, where the contents are broken down and recycled.
- Endocytosis: Lysosomes digest materials ingested from the extracellular environment via endocytosis. Phagosomes (from phagocytosis) and endosomes (from pinocytosis) fuse with lysosomes to degrade their contents.
Waste Removal:
- Breakdown of Cellular Debris: Lysosomes degrade cellular waste products, preventing accumulation of harmful substances and maintaining cellular homeostasis.
Defense Mechanism:
- Pathogen Destruction: In immune cells like macrophages and neutrophils, lysosomes digest engulfed pathogens such as bacteria and viruses, contributing to the body’s defense against infections.
Apoptosis (Programmed Cell Death):
- Caspase Activation: Lysosomal enzymes can be released into the cytoplasm to activate caspases, enzymes that execute apoptosis, aiding in controlled cell death.
Recycling of Cellular Components:
- Reuse of Molecules: Digested materials are transported out of lysosomes and reused for various cellular processes, conserving resources and energy.
Consequences of Lysosomal Dysfunction:
Lysosomal Storage Diseases:
- Definition: Genetic disorders caused by deficiencies in specific lysosomal enzymes, leading to the accumulation of undigested substrates.
- Examples:
- Gaucher Disease: Deficiency in glucocerebrosidase leads to the buildup of glucocerebroside in macrophages.
- Tay-Sachs Disease: Deficiency in hexosaminidase A results in the accumulation of GM2 gangliosides in neurons.
- Pompe Disease: Deficiency in acid alpha-glucosidase causes glycogen accumulation in muscle cells.
- Consequences: Accumulation of substrates disrupts cellular function, leading to organ enlargement, neurological symptoms, and impaired development.
Cellular Damage and Death:
- Accumulation of Waste: Undigested substrates can interfere with cellular processes, causing organelle dysfunction and impaired metabolism.
- Autophagic Blockage: Incomplete autophagy results in the buildup of damaged organelles, contributing to cellular stress and apoptosis.
- Apoptosis Induction: Excessive release of lysosomal enzymes into the cytoplasm can trigger uncontrolled cell death, impacting tissue integrity and function.
Neurodegenerative Diseases:
- Alzheimer’s Disease: Impaired lysosomal function is associated with the accumulation of amyloid-beta plaques and tau tangles.
- Parkinson’s Disease: Dysfunctional lysosomes contribute to the buildup of alpha-synuclein aggregates, affecting neuronal health.
Immune System Impairment:
- Reduced Pathogen Clearance: Defective lysosomes in immune cells hinder the digestion of engulfed pathogens, compromising the body’s ability to fight infections.
Cancer Progression:
- Tumorigenesis: Lysosomal dysfunction can contribute to cancer progression by affecting cellular signaling pathways, apoptosis, and autophagy, enabling cancer cells to evade death and sustain growth.
Mechanisms Preventing Lysosomal Dysfunction:
Proper Enzyme Targeting:
- Mannose-6-Phosphate Tagging: Lysosomal enzymes are tagged with mannose-6-phosphate in the Golgi apparatus, ensuring their delivery to lysosomes via mannose-6-phosphate receptors.
Quality Control Systems:
- ER-Associated Degradation (ERAD): Misfolded lysosomal enzymes are identified and targeted for degradation before they reach lysosomes.
- Chaperone Proteins: Assist in proper folding of lysosomal enzymes, preventing accumulation of non-functional proteins.
Autophagy Regulation:
- Efficient Autophagic Flux: Ensures timely digestion and recycling of cellular components, maintaining lysosomal health and function.
Conclusion:
Lysosomes are essential for cellular waste management, recycling, and defense mechanisms. Their dysfunction can lead to severe cellular and systemic consequences, including lysosomal storage diseases, neurodegeneration, immune impairment, and cancer progression. Understanding lysosomal biology is crucial for diagnosing and developing treatments for these conditions, highlighting the organelles’ pivotal role in maintaining cellular and organismal health.
10. How do tumor suppressor genes and oncogenes interact to regulate the cell cycle, and what happens when their functions are altered?
Answer:
Tumor Suppressor Genes and Oncogenes Overview:
Tumor suppressor genes and oncogenes are two categories of genes that play critical roles in regulating the cell cycle and maintaining cellular homeostasis. Their balanced interactions are essential for preventing uncontrolled cell proliferation and tumor formation.
1. Tumor Suppressor Genes:
Definition: Genes that encode proteins responsible for inhibiting cell division, repairing DNA, and inducing apoptosis. They act as brakes on the cell cycle.
Function:
- Cell Cycle Inhibition: Prevent progression to the next cell cycle phase when conditions are unfavorable.
- DNA Repair: Facilitate the correction of DNA damage, maintaining genomic integrity.
- Apoptosis Induction: Trigger programmed cell death in cells with irreparable damage.
Key Examples:
- TP53 (p53): Activates DNA repair proteins, induces cell cycle arrest via p21, and promotes apoptosis.
- RB1 (Retinoblastoma Protein): Inhibits E2F transcription factors, preventing G₁ to S phase transition.
- BRCA1/BRCA2: Involved in homologous recombination repair of double-strand breaks.
Alterations and Consequences:
- Loss of Function: Mutations, deletions, or epigenetic silencing of tumor suppressor genes reduce their inhibitory effects.
- Impact: Unregulated cell cycle progression, accumulation of DNA damage, and increased risk of cancer.
- Example: Mutation of TP53 is one of the most common alterations in human cancers, leading to impaired DNA damage response and apoptosis.
2. Oncogenes:
Definition: Genes that, when mutated or overexpressed, promote cell division, survival, and other processes that contribute to tumorigenesis. They act as gas pedals for the cell cycle.
Function:
- Cell Cycle Promotion: Enhance progression through cell cycle phases by activating cyclin-CDK complexes or downstream signaling pathways.
- Cell Survival and Growth: Support cellular proliferation and prevent apoptosis.
Key Examples:
- RAS: Encodes a GTPase involved in transmitting growth signals; mutations lead to constitutive activation.
- MYC: Transcription factor that drives expression of genes involved in cell growth and proliferation.
- HER2/neu (ERBB2): Receptor tyrosine kinase that, when overexpressed, promotes aggressive cell division.
Alterations and Consequences:
- Gain of Function: Mutations, gene amplification, or chromosomal translocations result in increased or unregulated activity.
- Impact: Enhanced cell proliferation, resistance to apoptosis, and increased potential for metastasis.
- Example: Amplification of HER2/neu in breast cancer leads to aggressive tumor growth and poor prognosis.
3. Interaction Between Tumor Suppressors and Oncogenes:
Balance of Signals: Tumor suppressor genes and oncogenes provide opposing signals that regulate cell cycle progression. A balance between these signals ensures controlled cell division.
Disruption of Balance: Alterations in either category can tip the balance towards uncontrolled proliferation and tumorigenesis.
- Loss of Tumor Suppressors + Activation of Oncogenes: Synergistically drive cancer progression by removing inhibitory controls and promoting proliferative signals.
- Example: Concurrent mutation of TP53 (loss of tumor suppressor) and activation of RAS (oncogene) significantly increases the likelihood of malignant transformation.
4. Mechanisms of Alteration:
- Tumor Suppressor Genes:
- Mutations: Point mutations, insertions, deletions that disrupt protein function.
- Gene Deletions: Loss of entire gene copies, reducing protein levels.
- Epigenetic Silencing: Methylation of promoter regions inhibits gene expression.
- Oncogenes:
- Point Mutations: Convert proto-oncogenes into constitutively active oncogenes.
- Gene Amplification: Increased copies lead to overexpression of the oncogenic protein.
- Chromosomal Translocations: Create fusion genes with enhanced or aberrant function.
5. Clinical Implications:
- Cancer Diagnosis and Prognosis:
- Genetic Profiling: Identifying mutations in tumor suppressors and oncogenes aids in diagnosing specific cancer types and predicting outcomes.
- Targeted Therapies:
- Oncogene Inhibition: Drugs like tyrosine kinase inhibitors target overactive oncogenes (e.g., HER2 inhibitors in breast cancer).
- Restoration of Tumor Suppressor Function: Although challenging, strategies aim to restore or mimic tumor suppressor activities.
- Personalized Medicine:
- Tailored Treatments: Based on the specific genetic alterations in a patient’s tumor, therapies can be customized for maximum efficacy.
Conclusion:
Tumor suppressor genes and oncogenes are fundamental regulators of the cell cycle, maintaining the balance between cell division and growth inhibition. Alterations in these genes disrupt this balance, leading to uncontrolled proliferation and cancer development. Understanding the interactions and regulatory mechanisms between tumor suppressors and oncogenes is essential for developing effective cancer diagnostics and targeted therapies, ultimately improving patient outcomes.
12. How do cell cycle inhibitors, such as p21 and p27, function to halt cell cycle progression, and what is their significance in cancer prevention?
Answer:
Cell Cycle Inhibitors Overview:
Cell cycle inhibitors, including p21 (Cip1/Waf1) and p27 (Kip1), are crucial regulators that halt cell cycle progression in response to various internal and external signals. They function by inhibiting cyclin-CDK complexes, thereby enforcing cell cycle checkpoints and preventing uncontrolled cell division.
1. Function of p21 and p27:
a. p21 (Cip1/Waf1): – Regulation by p53: – Response to DNA Damage: Activated p53, a tumor suppressor, induces the expression of p21 in response to DNA damage or stress signals. – Inhibition of Cyclin-CDK Complexes: – Binding: p21 binds to and inhibits Cyclin E-CDK2 and Cyclin D-CDK4/6 complexes, blocking progression from G₁ to S phase. – Effect on Rb Phosphorylation: Prevents Rb phosphorylation, maintaining Rb in its active state and inhibiting E2F-mediated transcription of S phase genes. – Role in Cell Cycle Arrest: – Temporary Halt: Allows cells time to repair DNA damage before resuming the cell cycle. – Apoptosis Induction: In cases of severe damage, p21 can contribute to apoptotic pathways by interacting with other regulatory proteins.
b. p27 (Kip1): – Regulation by Growth Signals: – Response to Growth Inhibition: p27 levels increase in response to anti-mitogenic signals, such as contact inhibition or differentiation cues. – Inhibition of Cyclin-CDK Complexes: – Binding: p27 inhibits Cyclin D-CDK4/6 and Cyclin E-CDK2 complexes, preventing G₁ to S phase transition. – Effect on Rb Phosphorylation: Maintains Rb in an active state, blocking E2F-mediated gene expression. – Role in Cell Cycle Control: – Differentiation: Promotes cell cycle exit during differentiation. – Senescence: Contributes to cellular senescence by maintaining cell cycle arrest.
2. Mechanism of Inhibition:
- Direct Binding: Both p21 and p27 directly bind to cyclin-CDK complexes, obstructing substrate access and inhibiting kinase activity.
- Stoichiometric Inhibition: High concentrations of p21/p27 relative to cyclin-CDK complexes effectively suppress their activity.
- Regulation by Proteolysis:
- Skp2-Mediated Degradation: The SCF^Skp2 ubiquitin ligase complex targets p27 for proteasomal degradation, allowing cell cycle progression. Regulation of Skp2 influences p27 levels and cell cycle control.
3. Significance in Cancer Prevention:
- Tumor Suppression:
- Enforcement of Checkpoints: By inhibiting cyclin-CDK activity, p21 and p27 enforce cell cycle checkpoints, preventing the propagation of damaged or abnormal cells.
- Prevention of Uncontrolled Proliferation: Halts cell division in response to DNA damage or oncogenic signals, reducing the risk of tumorigenesis.
- Genomic Integrity Maintenance:
- DNA Repair Facilitation: Cell cycle arrest allows time for DNA repair mechanisms to correct mutations and prevent genomic instability.
- Promotion of Apoptosis:
- Elimination of Damaged Cells: In cases where DNA damage is irreparable, p21 and p27 can aid in directing cells towards apoptosis, eliminating potentially cancerous cells.
- Inhibition of Metastasis:
- Cell Cycle Exit: By promoting cell cycle arrest, p21 and p27 reduce the proliferation rate of cancer cells, limiting tumor growth and metastasis.
- Clinical Implications:
- Prognostic Markers: Low levels of p21 and p27 are often associated with poor prognosis in various cancers, as their absence facilitates unchecked cell division.
- Therapeutic Targets: Strategies to restore or mimic p21/p27 function are being explored as potential cancer treatments, aiming to reinstate cell cycle control and inhibit tumor growth.
4. Examples of p21 and p27 in Cancer:
- p21 Deficiency:
- Increased Tumorigenesis: Mice lacking p21 exhibit higher rates of spontaneous and chemically-induced tumors.
- Human Cancers: Reduced p21 expression is observed in various cancers, including breast, colon, and lung, correlating with advanced disease stages.
- p27 Downregulation:
- Poor Prognosis: Low p27 levels are linked to aggressive tumor behavior and decreased survival rates in cancers like prostate, gastric, and ovarian.
- Mechanisms: Overexpression of Skp2, which targets p27 for degradation, is common in cancers and contributes to reduced p27 levels.
Conclusion:
Cell cycle inhibitors p21 and p27 are essential for maintaining proper cell cycle control, enforcing checkpoints, and preventing the proliferation of damaged or abnormal cells. Their ability to inhibit cyclin-CDK complexes makes them vital tumor suppressors. Dysregulation or loss of p21 and p27 function is frequently associated with cancer development and progression, underscoring their significance in cancer prevention and as targets for therapeutic intervention.
11. What are the roles of cell junctions in cell communication, and how do different types of junctions contribute to tissue integrity and function?
Answer:
Cell Junctions in Cell Communication:
Cell junctions are specialized structures that connect adjacent cells, facilitating communication and maintaining tissue integrity. They play critical roles in coordinating cellular activities, regulating the passage of substances between cells, and providing mechanical support to tissues.
Types of Cell Junctions and Their Functions:
Tight Junctions (Occluding Junctions):
- Structure: Composed of claudin and occludin proteins that form a seal between adjacent cells near their apical surfaces.
- Function:
- Barrier Formation: Prevent the free passage of molecules and ions through the space between cells, maintaining distinct extracellular environments.
- Apical-Basal Polarity: Help establish and maintain the polarity of epithelial cells by preventing the mixing of apical and basolateral membrane proteins.
- Location: Predominantly found in epithelial tissues lining organs and body cavities (e.g., intestinal epithelium, blood-brain barrier).
Adherens Junctions (Zonula Adherens):
- Structure: Comprised of cadherin proteins linked to the actin cytoskeleton via catenins.
- Function:
- Mechanical Attachment: Provide strong mechanical bonds between cells, maintaining tissue integrity.
- Signal Transduction: Involved in transmitting signals that regulate cell behavior and differentiation.
- Location: Common in epithelial and endothelial tissues, forming a belt-like structure encircling the cell.
Desmosomes (Macula Adherens):
- Structure: Consist of cadherins (desmogleins and desmocollins) linked to intermediate filaments (keratin) via adaptor proteins (desmoplakins and plakoglobins).
- Function:
- Mechanical Strength: Distribute tensile forces across tissues, preventing cells from tearing apart under stress.
- Tissue Integrity: Essential for maintaining the structural integrity of tissues subjected to mechanical stress, such as skin and cardiac muscle.
- Location: Abundant in tissues like the epidermis, heart muscle, and uterus.
Gap Junctions:
- Structure: Composed of connexin proteins that form channels (connexons) allowing direct cytoplasmic communication between adjacent cells.
- Function:
- Electrical and Chemical Coupling: Permit the passage of ions, metabolites, and signaling molecules, enabling coordinated cellular activities.
- Synchronization: Essential for synchronized muscle contractions and coordinated responses in neuronal networks.
- Location: Found in cardiac muscle, smooth muscle, neurons, and various epithelial tissues.
Hemidesmosomes:
- Structure: Similar to desmosomes but connect cells to the extracellular matrix instead of adjacent cells. Composed of integrins linked to intermediate filaments.
- Function:
- Anchoring: Secure epithelial cells to the underlying basement membrane, providing stability and resistance to shear stress.
- Location: Prominently present in epithelial tissues, such as the skin’s basal layer.
Anchoring Junctions:
- Includes: Desmosomes and hemidesmosomes.
- Function: Provide strong adhesion between cells and between cells and the extracellular matrix, ensuring tissue resilience.
Contribution to Tissue Structure and Function:
Mechanical Stability:
- Tissue Cohesion: Desmosomes and adherens junctions distribute mechanical forces across tissues, preventing cells from detaching or being damaged under stress.
- Structural Support: Gap junctions and tight junctions help maintain the organized structure of tissues by ensuring cells remain connected and aligned.
Barrier Function:
- Selective Permeability: Tight junctions regulate the movement of substances between cells, maintaining distinct environments on either side of epithelial layers (e.g., preventing leakage in the blood-brain barrier).
- Protection: By forming barriers, cell junctions protect underlying tissues from pathogens and harmful substances.
Cell Communication:
- Direct Cytoplasmic Exchange: Gap junctions allow cells to share ions and small molecules, facilitating coordinated responses and metabolic cooperation.
- Signal Transduction: Adherens junctions participate in signaling pathways that influence cell proliferation, differentiation, and migration.
Cellular Organization:
- Polarity Maintenance: Tight junctions help establish and preserve the polarity of epithelial cells, ensuring directional transport of materials (e.g., nutrient absorption in the intestines).
- Compartmentalization: Junctions contribute to the spatial organization of cells within tissues, essential for specialized functions.
Consequences of Cell Junction Dysfunction:
Loss of Tissue Integrity:
- Detachment and Fragility: Impaired desmosomes or hemidesmosomes can lead to weakened tissue cohesion, making tissues susceptible to injury and detachment (e.g., blistering skin diseases like pemphigus vulgaris).
Barrier Dysfunction:
- Leakage and Inflammation: Disrupted tight junctions can cause leaky barriers, allowing pathogens and toxins to enter tissues and trigger inflammatory responses (e.g., leaky gut syndrome).
Impaired Communication:
- Uncoordinated Activities: Defective gap junctions can lead to desynchronized cellular activities, affecting processes like heart contractions and neural signaling.
Cancer Progression:
- Metastasis: Loss of cell adhesion properties can facilitate cancer cell detachment and spread to other parts of the body.
- Tumor Growth: Impaired signaling through adherens junctions can contribute to uncontrolled cell proliferation.
Conclusion:
Cell junctions are fundamental to maintaining the structural integrity, selective permeability, and coordinated function of tissues in animal cells. By providing mechanical stability, regulating substance movement, and facilitating communication, they ensure the proper organization and operation of multicellular organisms. Dysfunction in cell junctions can lead to a range of diseases, highlighting their critical role in health and disease.
12. How do lysosomes and peroxisomes differ in their functions and contributions to cellular metabolism, and what are the implications of their dysfunction in human diseases?
Answer:
Lysosomes and Peroxisomes in Cellular Metabolism:
Lysosomes and peroxisomes are both membrane-bound organelles involved in cellular metabolism, but they perform distinct functions and contribute differently to cellular homeostasis.
Lysosomes:
Function:
- Intracellular Digestion: Lysosomes contain a variety of hydrolytic enzymes that break down all types of biological polymers, including proteins, nucleic acids, carbohydrates, and lipids.
- Autophagy: Lysosomes digest and recycle damaged or obsolete organelles and macromolecules within the cell.
- Endocytosis: Lysosomes degrade materials ingested from the extracellular environment via endocytosis.
- Defense Mechanism: In immune cells like macrophages and neutrophils, lysosomes digest engulfed pathogens.
Contribution to Cellular Metabolism:
- Waste Removal: Prevents accumulation of cellular waste products, maintaining cellular homeostasis.
- Recycling: Provides building blocks for new cellular components by breaking down macromolecules.
- Energy Production: Indirectly contributes by recycling nutrients that can be used in metabolic pathways.
Implications of Lysosomal Dysfunction:
- Lysosomal Storage Diseases: Genetic deficiencies in specific lysosomal enzymes lead to accumulation of undigested substrates, causing cellular and tissue dysfunction.
- Examples: Gaucher disease, Tay-Sachs disease, Pompe disease.
- Neurodegenerative Diseases: Impaired lysosomal function is associated with accumulation of toxic proteins in diseases like Alzheimer’s and Parkinson’s.
- Immune Impairment: Defective lysosomes reduce the ability of immune cells to clear pathogens, increasing susceptibility to infections.
- Lysosomal Storage Diseases: Genetic deficiencies in specific lysosomal enzymes lead to accumulation of undigested substrates, causing cellular and tissue dysfunction.
Peroxisomes:
Function:
- Fatty Acid Metabolism: Peroxisomes are involved in the β-oxidation of very-long-chain fatty acids, converting them into medium-chain fatty acids that can be further processed in mitochondria.
- Reactive Oxygen Species (ROS) Detoxification: Contain enzymes like catalase that break down hydrogen peroxide (H₂O₂), a reactive oxygen species, into water and oxygen.
- Bile Acid Synthesis: Play a role in the synthesis of bile acids from cholesterol.
- Detoxification of Harmful Substances: Metabolize toxic compounds, including ethanol and fatty alcohols.
Contribution to Cellular Metabolism:
- Lipid Metabolism: Essential for processing fatty acids and maintaining lipid homeostasis.
- Detoxification: Protects cells from oxidative damage by detoxifying reactive oxygen species and harmful substances.
- Biochemical Pathways: Participate in biosynthetic pathways necessary for cellular functions and signaling.
Implications of Peroxisomal Dysfunction:
- Peroxisomal Disorders: Genetic mutations affecting peroxisomal enzymes lead to metabolic dysfunctions.
- Examples: Zellweger syndrome, Adrenoleukodystrophy, Refsum disease.
- Lipid Accumulation: Defects in fatty acid metabolism result in the accumulation of very-long-chain fatty acids, which can disrupt cellular membranes and organelle function.
- Oxidative Stress: Impaired ROS detoxification leads to increased oxidative damage, contributing to aging and various diseases.
- Neurological Impairment: Accumulation of toxic lipids and oxidative damage in the nervous system can cause severe neurological deficits and developmental delays.
- Peroxisomal Disorders: Genetic mutations affecting peroxisomal enzymes lead to metabolic dysfunctions.
Differences Between Lysosomes and Peroxisomes:
Feature | Lysosomes | Peroxisomes |
---|---|---|
Primary Function | Intracellular digestion and recycling | Fatty acid metabolism and ROS detoxification |
Enzyme Content | Hydrolytic enzymes (proteases, lipases, nucleases) | Oxidative enzymes (catalase, acyl-CoA oxidases) |
Energy Metabolism | Indirect role through recycling | Direct role in lipid metabolism and energy production |
Reactive Oxygen Species | Limited detoxification via enzymes like catalase | Extensive detoxification of H₂O₂ and other ROS |
Biogenesis | Formed from the Golgi apparatus | Bud from the endoplasmic reticulum |
Implications of Dysfunction | Lysosomal storage diseases, neurodegeneration | Peroxisomal disorders, lipid accumulation, oxidative stress |
Conclusion:
Lysosomes and peroxisomes are vital organelles that contribute uniquely to cellular metabolism and homeostasis. Lysosomes focus on waste degradation and recycling, while peroxisomes handle lipid metabolism and detoxification of reactive oxygen species. Dysfunction in either organelle can lead to severe metabolic and neurological diseases, highlighting their essential roles in maintaining cellular and organismal health. Understanding their distinct functions and regulatory mechanisms is crucial for diagnosing and developing treatments for related disorders.
Conclusion:
These twelve thought-provoking questions and detailed answers cover essential aspects of the cell cycle, including its phases, regulation by cyclins and CDKs, role of checkpoints, mitosis and cytokinesis processes, key regulatory proteins like Rb and APC/C, the impact of dysregulation on cancer development, and the function of cell cycle inhibitors. They are designed to deepen understanding, promote critical thinking, and reinforce key concepts essential for mastering cell biology. Utilizing these questions as study aids can help learners grasp the complexities of cell cycle regulation and its implications in health and disease.